Abstract
Angiogenesis is an important process in cancer growth and metastasis. During the tumor angiogenic process, endothelial cells express various cell surface receptors which can be utilized for molecular imaging and targeted drug delivery. One such protein receptor of interest is the integrin αvβ3. Our group is involved in the development of molecular imaging probes and drug delivery systems targeting αvβ3. Based on extensive lead optimization study with the integrin antagonist compounds, we have developed a new generation of integrin αvβ3 compound (IA) which has superior binding affinity to αvβ3. Utilizing this IA as a targeting agent, we have developed a novel integrin-targeted nanoparticle (ITNP) system for targeted drug delivery and imaging of cancer angiogenesis. When multiple copies of the IA were conjugated onto the nanoparticle surface, strong and selective binding to the integrin αvβ3 was observed. These ITNPs also were rapidly taken up by cells that express αvβ3. The ITNPs accumulated in the angiogenic vessels, after systemic administration in a murine squamous cell carcinoma model. This novel intergrin targeted ITNP platform will likely have an application in targeted delivery of drugs and genes in vivo and can also be used for molecular imaging.
Introduction
Angiogenesis is required for cancer growth and is highly associated with cancer metastasis. Integrin αvβ3 is a cell-surface receptor protein that is upregulated in various pathological conditions including osteoporosis, rheumatoid arthritis, macular degeneration as well as cancer angiogenesis (CitationEliceiri and Cheresh 2000; CitationRuoslahti 2002). Integrin αvβ3 is a widely recognized target for the development of molecular probes for imaging angiogenesis and for cancer therapy. Integrin αvβ3 antagonists induce apoptosis on activated endothelial cells and also αvβ3 positive tumor cells (CitationTaga et al 2002). Vitaxin, an antibody for αvβ3 and cilengitide, a cyclic peptide (CitationTucker 2006) both block αvβ3 and are in clinical trials as antiangiogenic agents in patients with various tumors (CitationGutheil et al 2000; CitationRaguse et al 2004). A number of research groups have developed αvβ3 targeted nanomaterials for imaging and therapy (CitationWinter et al 2003; Lim et al 2006). A paramagnetic polymerized liposomal nanoparticle was developed by attaching the αvβ3 antibody LM609 onto the surface of the nanoparticle (CitationStorrs et al 1995), and this polymerized nanoparticle showed good tumor accumulation in the VX2 rabbit tumor model (CitationSipkins et al 1998). Similar targeted polymerized liposomes have also been developed for targeted gene delivery, in which the targeting agent is a small molecule integrin antagonist instead of an antibody (CitationHood et al 2002). These particles also demonstrated angiogenesis targeting in murine tumor models. We have developed a novel integrin antagonist which has more than 20 times better binding affinity to αvβ3 compared to the one that was used by Hood et al (CitationDuggan et al 2000; CitationBurnett et al 2005). Here we report a novel ITNP system that has this high affinity integrin antagonist compound as the targeting agent and the subsequent studies involving the in vivo tumor angiogenesis targeting.
Methods and materials
Synthesis of targeting lipid BisT-PE-EDTA-IA ()
Ethylenediaminetetraacetic acid dianhydride (EDTA-dianhydride, 135 mg, 525 μ moles) was dissolved in a dry 3-neck flask, under a positive pressure of argon, in pyridine (20 mL). To this solution was added 1,2-bis(10,12-tricosadiynoyl)-sn-glycero-3-phosphoethanolamine (436 mg, 500 μmoles) in methylene chloride (5 mL) drop wise using a syringe pump at the rate of 2.5 mL per hour. The starting material had completely disappeared after two hours stirring, as observed by thin layer chromatography. To this reaction was added the integrin antagonist compound (271mg, 550 μmoles) and allowed to stir for 72 hours. The reaction mixture was partitioned between methylene chloride (100 mL) and water (20 mL). The organic layer was separated and washed with brine (10 mL) and then dried over anhydrous sodium sulfate. The solution was filtered to remove sodium sulfate and the solvent was then removed by spin evaporation and the residue was dried under high vacuum over night. The crude product was purified by flash chromatography using CHCl3/CH3OH/H2O/NH4OH (50/40/5/5) as the solvent to yield 102 mg of the desired product (yield = 13%). 1H NMR: (CDCl3:CD3OD 1:1, 300 MHz, δ ppm) 7.65 (2H, d, Ar-H), 6.75 (2H, d, Ar-H), 0.65–1.05 (116H, overlapping peaks, 2-CH3 54-CH2 and 2-CH), 0.8 (6H, s, CH3). MALDI-MS: M+H+ = 1585.842.
Synthesis of integrin-targeted nanoparticle (ITNP)
The ITNP consists of two lipids with encapsulated rhodamine-B (). The first lipid is 1,2-bis(10,12-tricosadiynoyl)-sn-glycero-3-phosphoethanolamine-ethylendiaminetetraacetic acid-integrin antagonist (BisT-PE-EDTA-IA) and the second lipid is the base lipid 1,2-bis(10,12-tricosadiynoyl)-sn-glycero-3-phosphocholine (BisT-PC) lipid. The BisT-PC lipid was purchased from Avanti Polar Lipids. The BisT-PE-EDTA-IA was synthesized from commercially available BisT-PE lipid as described above. Targeting lipid (BisT-PE-EDTA-IA) and the base lipid (BisT-PC, Avanti lipids) were dissolved in a 1:1 mixture of chloroform and methanol and were spin-evaporated to make a thin film. The film was dried under high vacuum over night. This film was hydrated with 10 mM ammonium chloride solution and was gently mixed. The suspension was subjected to 5 consecutive freeze-thaw cycles followed by 5 extrusion cycles through a 100 nm pore size polycarbonate membrane. The extruded particles were then dialyzed against a solution of rhodamine-B for 2 hours. The rhodamine-B loaded ITNPs were then dialyzed against 10 mM HEPES buffer pH 7.4 for 3 hours. The dialyzed ITNPs were then filtered through 0.2 μ filter into a sterile vial. The size and zeta potential of the ITNPs were determined by dynamic light scattering. The fluorescence spectra was recorded for the rhodamine.
Figure 1 (a) Schematic diagram outlining the formation of the ITNPs by self-assembly of the appropriate lipids. The lipid with targeting agent (10 mole%) was combined with base lipid (90 mole%) in a solution of chloroform and methanol (1:1). The mean diameter of the ITNPs was 104.4 ± 3.3 nm, as determined by dynamic light scattering, and the zeta potential was approximately −43.8 mv. The ITNPs were stable for weeks without any observable changes in their physical and biological properties when formulated for use in vivo. (b) Schematic diagram outlining the formation of the Control-NPs by self-assembly of the appropriate lipids. The mean diameter of the nanoparticle was 101.2 ± 2.4 nm, as determined by dynamic light scattering, and the zeta potential was approximately −32.6 mv.
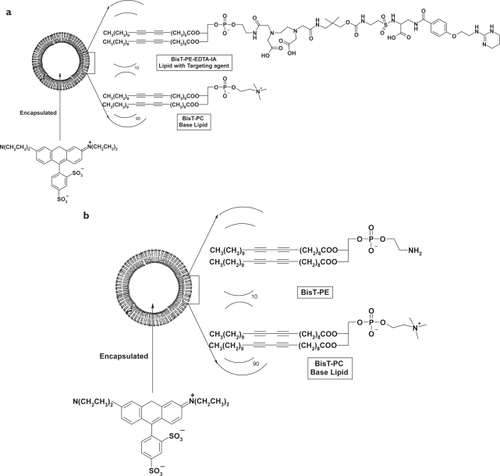
Synthesis of control-NP
The control-NP consists of two lipids with encapsulated rhodamine-B. BisT-PE and BisT-PC lipids (Avanti Polar lipids) were dissolved in a 1:1 mixture of chloroform and methanol and were spin-evaporated to make a thin film. The film was dried under high vacuum over night. This film was hydrated with 10 mM ammonium chloride solution and was gently mixed. The suspension was subjected to 5 consecutive freeze-thaw cycles followed by 5 extrusion cycles through a 100 nm pore size polycarbonate membrane. The extruded particles were then dialyzed against a solution of rhodamine-B for 2 hours. The rhodamine-B loaded NPs were then dialyzed against 10 mM HEPES buffer pH 7.4 for 3 hours. The dialyzed control-NPs were then filtered through 0.2 μ filter into a sterile vial. The size and zeta potential of the control-NPs were determined by dynamic light scattering.
Transmission electron microscopy of the ITNPs
20 μl of the ITNP was added onto a carbon coated Formvar-film grid. The excess solution was removed by blotting and to this was added 1% phosphotungstic acid solution, pH 7.0. Excess phosphotungstic acid was removed by blotting. The grid surface was then air dried at room temperature. The grid was examined by an electron microscope operated at 75 kV (Hitachi H7000, Tokyo). Digital images were taken by a charge-coupled device camera (Gatan, Pleasanton, CA).
Binding affinity assay
Purified integrin αvβ3 protein (Chemicon International, Temecula, CA) was applied to 96-well polystyrene microtiter plates at 0.1 μg/well. After overnight incubation at 4 °C the plates were washed, and then blocked with milk solution (KPL, Inc., Gaithersburg, MD) at room temperature for 2 h. The blocking buffer was removed, and the plates were inoculated in quadruplicate with ITNPs with a typical starting concentration of 1.25 μM. Serial dilutions were prepared in the 96-well plates using multichannel pipette. To each well was added biotinylated vitronectin solution (0.1 μg/well) as standard competitor. The plates were incubated at room temperature for 3 h, washed, and the bound vitronectin was detected using NeutrAvidin-HRP conjugate at 0.01 μg/well (Pierce, Rockford, IL) and LumiGlo chemiluminescent substrate system (KPL, Inc., Gaithersburg, MD). The luminescence was read using a Wallac Counter. The concentration of inhibitor producing 50% inhibition (IC50) of vitronectin binding to αvβ3 was calculated based on a curve fitting model (CitationBurnett et al 2005).
Cell uptake assay of the ITNPs
The M21 cells were cultured in RPMI 1640 medium (Biofluids, Gaithersburg, MD) containing 10% fetal calf serum, 2 mM L-glutamine, and penicillin-streptomycin (50 IU ml−1 and 50 μg ml−1, respectively, BioSource International; Rockville, MD) at 37 °C with 5% CO2, and were continuously maintained without over-confluences. Before the experiment, the cells were passaged at 60%–70% confluence two to three times and the cells were loaded to the glass culture chambers for the ITNPs cell uptake assay. Two hours before the cell tracking experiment cells were transferred into fresh medium to ensure the good condition of the cells. The ITNPs were added to the chamber yielding a final concentration of 12 μmol/ml in the presence of 10% fetal bovine serum culture medium for 5 min, 15 min, and 30 min separately to obtain time course of the uptake of ITNPs into the cells. At different time points, the cells in the culture chamber were washed and fixed with 4% Paraformaldehyde-phosphate buffered saline for 20 minutes at RT. After fixing, the cells were washed again and counterstained with a solution of 4′-6-Diamidino-2-phenylindole (DAPI) and observed by the Fluorescence microscopy to obtain the cellular distribution of the ITNPs at different time points.
In vivo/ex vivo studies
A SCC 7, murine squamous cell carcinoma model was used for the in vivo studies. The cells were cultured in RPMI 1640 medium (Biofluids, Gaithersburg, MD) containing 10% fetal calf serum, 2 mM L-glutamine, and penicillin-streptomycin (50 IU ml−1 and 50 μg ml−1, respectively, BioSource International, Rockville, MD) at 37 °C with 5% CO2. The cells were pre-tested as mycoplasm negative (Research Animal Diagnostic Laboratory, University of Missouri, MO). Female C3H mice (6–9 weeks) were purchased from Charles River Laboratories (Gaithersburg, MD) to establish the squamous cell carcinoma mouse model. All animal work was performed according to an approved animal protocol and in compliance with the NIH Clinical Center Animal Care and Use Committee guidelines. Prior to the implantation of the tumor cells, the mice flanks were shaved bilaterally and prepped with isopropyl alcohol. A suspension of SCC-7 cells (1 × 106 cells in 100 μl of PBS) was injected in each of the flanks of each mouse with a 27 G needle. On average, a tumor required 10 days to grow to half centimeter before the mice received the tail-vein injection of Rhodamine-B encapsulated ITNPs. Each mouse received 100 μl of the ITNPs, and after 2 hrs the animal was euthanized. However, 15 minutes before euthanization, the mouse was injected with fluorescein isothiocyante-lectin (FITC-lectin) by tail vein (CitationHunter et al 2006). The tumor was harvested for cryostat sections. The slides were counterstained with DAPI and the slides were examined with a Zeiss upright microscope using Rhodamine, FITC and DAPI filters to determine the biodistribution of the ITNPs within the cancerous tissues.
Results and discussion
Assembly of integrin-targeted ITNPs
For the construction of the integrin-targeted ITNPs, the critical step is the synthesis of targeting lipid BisT-PE-EDTA-IA as shown in . The IA was synthesized following previously published procedure (CitationBurnett 2005). IA contains a primary amine functionality for the synthesis of the targeting lipid. EDTA-dianhydride has two reactive anhydrides and can be conjugated to two different amines one on each side by a single pot reaction. The IA and BisT-PE lipid each have one primary amine and by controlled reaction conditions we were able to conjugate them with EDTA-dianhydride. The crude product was purified by column chromatography. The product was characterized by NMR and mass spectrometry. The ITNPs consists of a targeting lipid and a base lipid. These two lipid solutions were mixed together and a film was made by evaporating the solvents. The film was hydrated and after few freeze-thaw cycles, the ITNPs were prepared by extrusion through a polycarbonate membrane. Rhodamine-B was encapsulated inside the particle by ion-exchange process. The particles were purified by dialysis and filtered through 0.2 μm filter into a sterile vial. The mean diameter of the ITNP was 104.4 ± 3.3 nm by dynamic light scattering, the pH was 7.40 and the zeta potential was −43.8 mV. An aliquot of the ITNPs when filtered through 10,000 MW cutoff spin filter, did not show any fluorescence in the filtrate indicating that rhodamine-B was stably encapsulated inside the ITNPs and did not leak out. The ITNPs were stained with phosphotungstic acid at pH 7.0 and examined with Transmission electron microscopy (TEM). The ITNPs appeared as vesicles formed by a single large aqueous cavity surrounded by a thick membrane (). The TEM also confirmed the size of ITNP was around 100 nm, in accordance with the measurement by dynamic light scattering.
Binding affinity and cellular uptake of the ITNPs
The binding affinity of the targeted ITNPs to αvβ3 was tested by coating 96 well plates with αvβ3. This is a competitive inhibition assay, where the ability of the ITNPs to competitively inhibit the binding of vitronectin to αvβ3. We found that ITNPs showed excellent binding affinity to the integrin αvβ3 (9.2 nM) (). The control nanoparticles did not bind to αvβ3 even at very high concentration in the integrin αvβ3 plate binding assay (data not shown) which is easy to understand that the bilayer lipids do not have a good binding affinity to the αvβ3 protein. Since we did not observe the binding affinity of non-targeted particles to the αvβ3 protein, therefore we specifically only observed ITNPs cell uptake and in vivo anagiogenesis targeting as following.
Figure 3 ITNPs binding affinity to the integrin α vβ 3 protein was quantified by the αvβ3 plate binding assay, as in method description. The concentration of inhibitor producing 50% inhibition (IC50) of vitronectin binding to αvβ3 was calculated based on a curve fitting model using KaleidaGraph 3.5 (Synergy Software, Reading, PA). ITNPs and targeting agent IA have a closed binding affinity to the αvβ3 protein.
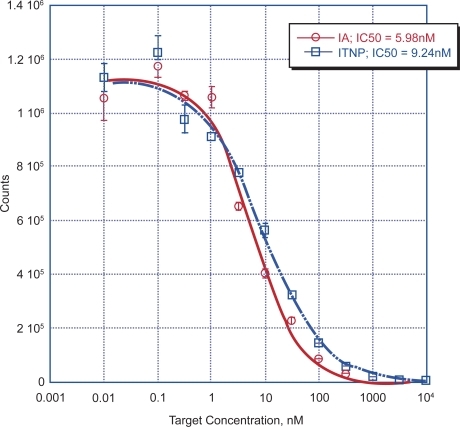
Cell uptake of the ITNPs
When ITNPs were incubated with the M21 cells, it was observed that the ITNPs were accumulated in the cells within minutes and major presented in the cytoplasm of the M21 cells, as observed by fluorescence microscopy (). This quick accumulation possibly happens by receptor mediated endocytosis. The uptake of the ITNPs was further investigated by live cell imaging and has been disclosed (CitationZhang et al 2006).
Tumor angiogenesis targeting
The targeted ITNPs were tail vein injected into C3H mice bearing murine squamous cell carcinoma (SCC-7) tumors to determine the in vivo ability to selectively target angiogenic vessels. Fifteen minutes prior to euthanasia, the animal was tail vein injected with FITC-lectin, which allowed us to observe the functional angiogenic vessels (CitationHunter 2006) and correlate with the distribution of the ITNPs in the functional angiogenic vessels. The ITNPs were visualized by fluorescence microscopy in the tumor angiogenic vessels which overlapped with the FITC-lectin signal, clearly demonstrating the angiogenesis specific targeting of these ITNPs (). Some accumulation was also seen in various other tissues, such as liver and spleen and minimal accumulation was observed in the lung capillary vessels, which warrants further optimization of the ITNPs such as size, charge and lipid composition.
Figure 5 Angiogenic vessel targeting by the ITNPs. A: SCC-7 Cell nucleus stained by DAPI, B: Functional angiogenic vessels stained by injected FITC-Lectin, C: angiogenic vessels targeting by ITNPs observed by Rhodamine, D: Merge signals of the ITNPs targeted vessels and functional angiogenic vessels labeled by Lectin, E: Merge signals of the ITNPs targeted vessels, functional angiogenic vessels labeled by Lectin, and cell nuclear staining by DAPI. (scale bar = 50 um).

Conclusion
We have synthesized a novel integrin targeted rhodamine-B encapsulated ITNP. This ITNP binds to the integrin αvβ3 in the in vitro assay. In vivo the ITNP targets the tumor angio-genic vessels, specially localized within the endothelial cells. Further optimization of this ITNP is feasible by modifying the size, charge and lipid composition. Incorporation of a wide variety of payloads for multi-modality imaging (magnetic resonance imaging, Positron emission tomography and optical imaging) and/or therapy (cytotoxic agent, radionu-clide, and anti vascular agents) is in progress.
Acknowledgements
We would like to thank Dr. David Cheresh of Scripps Research Institutes, La Jolla, CA for kindly providing the M21 cells. The SCC 7 cells were kindly provided by Dr. Anastasia Sowers, Radiation Oncology Branch, National Cancer Institute, Bethesda, MD. We also would like to thank Dr. Kunio Nagashima of National Cancer Institute for his help with the TEM. Special thanks go to Dr. Dale Kiesewetter and Dr. L. Henry Bryant for their help with this manuscript. The intramural research program of the NIH Clinical Center supported this work.
References
- BurnettCAXieJQuijanoJ2005Synthesis, in vitro, and in vivo characterization of an integrin alpha(v)beta(3)-targeted molecular probe for optical imaging of tumorBioorg Med Chem1337637115863003
- DugganMEDuongLTFisherJE2000Nonpeptide alpha(v)beta(3) antagonists. 1. Transformation of a potent, integrin-selective alpha(IIb)beta(3) antagonist into a potent alpha(v)beta(3) antagonistJ Med Chem4337364511020288
- EliceiriBPChereshDA2000Role of alpha v integrins during angiogenesisCancer Journal6S2459
- GutheilJCCampbellTNPiercePR2000Targeted antiangiogenic therapy for cancer using Vitaxin: a humanized monoclonal antibody to the integrin αvβ3Can Res6117815
- HoodJDBednarskiMFraustoR2002Tumor regression by targeted gene delivery to the neovasculatureScience2962404712089446
- HunterFXieJTrimbleC2006Rhodamine-RCA in vivo labeling guided laser capture microdissection of cancer functional angiogenic vessels in a murine squamous cell carcinoma mouse modelMol Can5111
- LimEHDanthiSNBednarskiM2005A review: Integrin avb3-targeted molecular imaging and therapy in angiogenesisNanomedicine: Nanotech Biol Med111014
- RaguseJDGathHJBierJ2004Cilengitide (EMD 121974) arrests the growth of a heavily pretreated highly vascularised head and neck tumourOral Oncol402283014693249
- RuoslahtiE2002Specialization of tumour vasculatureNat Rev Cancer2839012635171
- StorrsRWTropperFDLiHY1995Paramagnetic polymerized liposomes: synthesis, characterization, and applications for magnetic resonance imagingJ Am Chem Soc2873016
- SipkinsDAChereshDAKazemiMR1998Detection of tumor angiogenesis in vivo by alphaVbeta3-targeted magnetic resonance imagingNat Med462369585240
- TagaTSuzukiAGonzalez-GomezI2002Integrin antagonist EMD 121974 induces apoptosis in brain tumor cells growing on vitronectin and tenascinInt J Cancer98690711920637
- TuckerGC2006Integrins: molecular targets in cancer therpayCur Oncol Reports896103
- WinterPMMorawskiAMCaruthersSD2003Molecular imaging of angiogenesis in early-stage atherosclerosis with αvβ3-integrin-targeted nanoparticlesCirculation1082270414557370
- ZhangTDanthiSNXieJ2006Live cell imaging of the endocytosis and the intracellular trafficking of multifunctional lipid nanoparticlesProc SPIE NanoBiophotonics Biomedical Appln36095