Abstract
Background
Polyamidoamine (PAMAM) and polypropylenimine (PPI) dendrimers are the commercially available and most widely used dendrimers in pharmaceutical sciences and biomedical engineering. In the present study, the loading and release behaviors of generation 3 PAMAM and generation 4 PPI dendrimers with the same amount of surface amine groups (32 per dendrimer) were compared using phenylbutazone as a model drug.
Methods
The dendrimer-phenylbutazone complexes were characterized by 1H nuclear magnetic resonance and nuclear Overhauser effect techniques, and the cytotoxicity of each dendrimer was evaluated.
Results
Aqueous solubility results suggest that the generation 3 PAMAM dendrimer has a much higher loading ability towards phenylbutazone in comparison with the generation 4 PPI dendrimer at high phenylbutazone-dendrimer feeding ratios. Drug release was much slower from the generation 3 PAMAM matrix than from the generation 4 PPI dendrimer. In addition, the generation 3 PAMAM dendrimer is at least 50-fold less toxic than generation 4 PPI dendrimer on MCF-7 and A549 cell lines.
Conclusion
Although the nuclear Overhauser effect nuclear magnetic resonance results reveal that the generation 4 PPI dendrimer with a more hydrophobic interior encapsulates more phenylbutazone, the PPI dendrimer-phenylbutazone inclusion is not stable in aqueous solution, which poses a great challenge during drug development.
Introduction
Dendrimers are synthetic macromolecules with hyperbranched structures, well defined ellipsoidal or globular shapes, and precise molecular weights.Citation1,Citation2 In comparison with traditional linear polymers, dendrimers have shown the following advantages when they are used as drug carriers: high drug loading ability endowed by the large numbers of surface functionalities and interior cavities;Citation3,Citation4 high bioavailability of drugs covalently or noncovalently attached to dendrimers because of the high penetration ability of dendrimers across cell membranes and biological barriers;Citation5 reproducible pharmacodynamic and pharmacokinetic behavior of dendrimer-based drug formulations due to their well defined structure and extremely low polydispersity;Citation6 multifunctional scaffolds for both targeted diagnosis and therapy given that the dendrimer can be easily functionalized with various bioactive moieties;Citation7–Citation9 and stable monomolecular micelles in physiological conditions which avoid the disassembly of amphiphilic polymeric micelles below their critical micelle concentrations.Citation1 Integrating these versatile features into a single molecule, dendrimers have become competitive candidates as drug carriers in the pharmaceutical industry.Citation10
Up to now, the polyamidoamine (PAMAM) dendrimer reported by Tomalia in 1985 and polypropylenimine (PPI) dendrimer synthesized by Meijer et al in 1993 are the most investigated dendrimers in the fields of drug delivery ().Citation11,Citation12 These two commercially available dendrimers were synthesized by a divergent strategy, in which the construction of dendrimer takes place in a stepwise manner by coupling repeated units to a central core, providing a series of radically concentric layers called “generations”.Citation1 Structurally, PAMAM dendrimer was synthesized by Michael addition of amine with acrylic acid methyl ester, followed by the aminolysis of the resulting ester by ethylene diamine to create new reaction sites for further Michael additions.Citation11 Similarly, PPI dendrimer was initiated by the Michael addition of amine with acrylonitrile and followed by the reduction of nitrile groups to yield primary amine groups which provides new branching points.Citation12
PAMAM and PPI dendrimers have excellent aqueous solubility which ensures stable aqueous dispersion of PAMAM-based and PPI-based drug formulations.Citation1 Full-generation PAMAM and PPI dendrimers are usually terminated with primary amine groups (pKa about 10.0), and the cationic surface of PAMAM and PPI can bind a large amount of negatively charged drugs through electrostatic interactions. Citation13 Low-generation PAMAM and PPI dendrimers have an open structure with an ellipsoidal shape and inner pockets which can be used to encapsulate hydrophobic drugs.Citation14,Citation15 The predominant difference in the structure of PAMAM and PPI is their interior pockets, ie, PAMAM has relatively polar pockets consisting of alkyl chain, tertiary amine, and amido groups, while PPI possesses nonpolar pockets consisting of alkyl chain and tertiary amine groups.Citation16 In addition, the length of branching units for PAMAM (seven bonds) is different from that of PPI dendrimer (four bonds), indicating that the size of PAMAM dendrimer is much larger than that of PPI dendrimer with an equivalent number of surface amine groups. These structural differences may have interesting physicochemical implications for the host behavior of PAMAM and PPI dendrimers. The binding constants of dendrimer and phenol blue are an order of magnitude larger for PPI versus PAMAM with the same amount of surface groups, indicating fewer polar pockets of PPI dendrimer.Citation16 Dendrimer with a PPI core and a PAMAM shell encapsulates hydrophobic pyrene molecules in the PPI layers rather than in the PAMAM layers.Citation17 Also, PAMAM and PPI dendrimers exhibited distinct behaviors in their ionic interactions with negatively charged guests, such as vitamins C, B3, and B6.Citation18 In a separate study, isobutyramide-terminated PPI dendrimer was found to be more hydrophobic than PAMAM dendrimer with an equivalent number of isobutyramide groups,Citation19 probably due to the higher density of surface groups and the more hydrophobic interior of PPI dendrimer.
In our previous studies, we have demonstrated that PAMAM dendrimers are able to solubilize different families of hydrophobic drugs.Citation20 In comparison with interior encapsulation, surface ionic interaction was found to be the major factor on the solubilization behavior of PAMAM dendrimer.Citation3,Citation13 The host behaviors of PAMAM dendrimer towards a list of drugs were analyzed by 1H nuclear magnetic resonance (NMR) titrations,Citation21 nuclear Overhauser effect spectroscopy (NOESY),Citation3 and pulsed-field gradient NMR.Citation22 The release of drugs from the PAMAM dendritic matrix depends on dendrimer generation and surface functionality, ionic strength, pH conditions, and the solvent.Citation21 PAMAM dendrimer exhibits low biocompatibility in A549 and MCF-7 cell lines, and the removal of surface charges on PAMAM dendrimer by acetylation can completely neutralize the cytotoxic activity of dendrimer on these cells.Citation23 All these studies suggest that the surface amine groups on PAMAM dendrimers are of central importance in the binding, release, and biocompatibility of this polymeric nanocarrier. Furthermore, PPI dendrimers were also able to enhance the solubility of hydrophobic drugs, increase their stability, and prolong their delivery in different routes.Citation24 However, systematic comparisons on the loading and release behaviors of PAMAM and PPI dendrimer as well as their complex structures with drugs are limited.
In this present study, we focused on comparing PPI and PAMAM dendrimers in five aspects, ie, drug loading efficiency, host-guest chemistry, complex stability, in vitro drug release behavior, and cytotoxicity. It should be noted that PPI and PAMAM dendrimers having the same number of surface amine groups do not share the same dendrimer generation. For example, both generation 4 PPI and generation 3 PAMAM dendrimers have 32 amine groups on their peripheries. Here, they were chosen as model dendrimers to compare the host behaviors of PPI and PAMAM dendrimers. Phenylbutazone was used as a model drug.
Materials and methods
Materials
Generation 4 diaminobutane-cored and amine-terminated PPI dendrimer was purchased from Sigma-Aldrich (St Louis, MO). Generation 3–5 ethylenediamine-cored and amine-terminated PAMAM dendrimer was purchased from Dendritech Inc (Midland, MI). Phenylbutazone was purchased from Shangqiu Tiankang Fine Chemical Co, Ltd (Henan, China). Methanol of high-performance liquid chromatography (HPLC) grade was purchased from Fisher Scientific (Fair Lawn, NJ). Acridine orange and ethidium bromide were gifts from the School of Life Sciences, East China Normal University. Deuterium oxide (D2O) was obtained from Beijing Chongxi High-Tech Incubator Co, Ltd (Beijing, China). Generation 3 PAMAM dendrimer was stored in methanol and the solvent was distilled before use. PAMAM and PPI dendrimers were prepared in aqueous solutions at a concentration of 10 mg/mL, which were used as stock solutions. The dendrimers and the other chemicals were used as received without further purification.
Phase solubility test
The solubilities of hydrophobic drugs such as phenylbutazone in PAMAM and PPI dendrimer solutions were conducted using the Higuchi–Connors method as described elsewhere.Citation25 A 2 mg sample of phenylbutazone was added into 1.5 mL Eppendorf microtest tubes before the drug solubility test, followed by addition of PAMAM and PPI dendrimer solutions at different concentrations (dendrimer concentration ranges from 0.07 to 0.36 mM). The dendrimer-drug suspensions were shaken (300 rpm) at room temperature for 24 hours to obtain a saturated phenylbutazone solution, and the mixtures were then centrifuged twice at 10,000 rpm for 5 minutes to remove undissolved drugs. The drug concentrations in the supernatants were analyzed using an HPLC method.
Gradient-feed method
A 10 mg/mL phenylbutazone solution was prepared by dissolving the drug in methanol. Drug solutions (40 μL) were added into Eppendorf microtest tubes, and the solvents in the tubes were removed to obtain phenylbutazone powder (0.4 mg in each tube). Herein, we used a gradient-feed method to compare the drug-loading abilities of PAMAM and PPI dendrimers. Generally, 4 mL of PAMAM or PPI solution (0.14 mM) was added into a microtest tube containing 0.4 mg phenylbutazone. The tube was then sonicated for 2 hours to ensure the drugs were dissolved in the dendrimer solution, followed by transfer of the mixture into a second tube with the same amount of phenylbutazone, and sonication of the dendrimer-drug mixture. The procedures were repeated until the solution was transferred into the twentieth tube. In each time interval, 10 μL of the supernatant from the dendrimer-drug mixture was withdrawn from the tubes and analyzed by an HPLC method to determine the drug concentrations in the dendrimer solutions. Three repeats were conducted for each sample.
HPLC method
The samples were analyzed using a reverse-phase HPLC instrument (Agilent 1200, Agilent, Chicago, IL) with a C18 column (4.6 mm diameter, 150 mm length, 5 μm particle size, Zorbax Eclipse XDB, Agilent) at 25°C. The mobile phase was methanol and water at a ratio of 55:45 with a flow rate of 1.0 mL/minute. A volume of 10 μL sample solution was injected and the phenylbutazone was detected at a wavelength of 264 nm. The retention time of phenylbutazone is 3.8 ± 0.3 minute. Phenylbutazone solutions (methanol) at different concentrations were prepared to obtain a standard curve for the drug. The drug concentration correlated linearly with the peak area in HPLC ranges from 0.0005 to 0.01 mg/mL (R = 0.9991).
1H NMR and NOESY analysis
1H NMR and two-dimensional NOESY experiments were obtained on a Bruker Advance 500.132 mHz NMR spectrometer at 298.2 ± 0.2 K for dendrimers and dendrimer-drug complexes (0.57 mM generation 3 PAMAM or generation 4 PPI and 18 mM phenylbutazone in D2O).
Chemical shift assignments of the proton peaks in dendrimers and dendrimer-drug complexes are listed as follows. 1H NMR for PPI dendrimer: 1.60 ppm (120H, br, –NCH2 CH2CH2N–, HA); 2.44 ppm (116H, br, –NCH2CH2CH2N–, HB); 2.48 ppm (64H, br, –NCH2CH2CH2NH2, HB′); 2.60 ppm (64H, br, –NCH2CH2CH2NH2, HC).
1H NMR for PAMAM dendrimer: 2.39 ppm (120H, br, –NCH2CH2CONH–, Ha); 2.60 ppm (56H, br, –CONH CH2CH2N–, Hb); 2.69 ppm (120H, br, –NCH2CH2CONH–, Hc); 2.79 ppm (64H, br, –CONHCH2CH2NH2, Hb′); 3.21 ppm (56H, br, –CONHCH2CH2N–, Hd); 3.26 ppm (64H, br, –CONHCH2CH2NH2, Hd′).
1H NMR for PPI–phenylbutazone complex: 0.86 ppm (3H, br, –CH2CH3, H8); 1.29 ppm (2H, br, –CH2CH2CH3, H7); 1.41 ppm (2H, br, –CH2CH2CH2–, H6); 1.57 ppm (120H, br, –NCH2CH2CH2N–, HA); 2.14 ppm (2H, br, –CH–CH2–CH2–, H5); 2.30 ppm (116H, br, –NCH2CH2CH2N–, HB); 2.38 ppm (64H, br, –NCH2CH2CH2NH2, HB′); 2.63 ppm (64H, br, –NCH2CH2CH2NH2, HC); 7.07 ppm (2H, br, Ar, H1); 7.24 ppm (4H, br, Ar, H3); 7.29 ppm (4H, br, Ar, H2).
1H NMR for PAMAM–phenylbutazone complex: 0.85 ppm (3H, br, –CH2CH3, H8); 1.28 ppm (2H, br, –CH2CH2CH3, H7); 1.40 ppm (2H, br, –CH2CH2CH2–, H6); 2.14 ppm (2H, br, –CH–CH2–CH2–, H5); 2.30–2.35 ppm (120H, br, –NCH2 CH2CONH– Ha); 2.50 ppm (56H, br, –CONHCH2CH2N–, Hb); 2.72 ppm (120H, br, –NCH2CH2CONH–, Hc); 2.89 ppm (64H, br, –CONHCH2CH2NH2, Hb′); 3.19 ppm (56H, br, –CONHCH2CH2N–, Hd); 3.31 ppm (64H, br, –CONH CH2CH2NH2′ Hd′); 7.08 ppm (2H, br, Ar, H1); 7.25 ppm (8H, br, Ar, H2,3).
For the two-dimensional NOESY experiment, 300 msec was chosen as the mixing time for the optimization of cross-peak intensities with minimum distortions during the period for NOE establishment. A certain amount of ethanol was added to the dendrimer-drug solutions as an internal standard. A relaxation delay of one second, an acquisition time of 213 msec, and a 90° pulse width of 7.7 μ were used. Sixteen transients were collected over 800 complex points in the t1 dimension. The data were processed by NMR Pipe software on a Linux system with standard Lorents-Gauss window function and zero filling in both dimensions. All data were shown with Sparky software.
In vitro drug release studies
The in vitro release behavior of phenylbutazone from PAMAM or PPI dendrimer matrixes in aqueous solutions was investigated. Dendrimer-phenylbutazone complexes were prepared by dissolving 1 mg drug in 2 mL 0.14 mM generation 3 PAMAM or generation 4 PPI dendrimer solutions. The dendrimer-drug mixture was sonicated for 2 hours and transferred into a dialysis bag with a molecular weight cutoff of 1000 Da, which was immediately immersed into 50 mL distilled water. The molecular weights for generation 3 PAMAM and generation 4 PPI dendrimers are 6900 Da and 3513 Da, respectively. The dendrimers and dendrimer- drug complexes were kept inside the dialysis bag while the free phenylbutazone molecules with a molecular weight of 308 Da released into the outer phase of the dialysis bag. At specific time intervals, 30 μL samples were withdrawn from the outer phase which was replenished with 30 μL distilled water. The phenylbutazone concentrations in the samples were analyzed using the HPLC method. Three repeats were conducted for each sample.
Cell culture and cytotoxicity assay
MCF-7 and A549 cells (American Type Culture Collection, Manassus, VA) were incubated at 37°C in 5% CO2 and Dulbecco’s modified Eagle’s medium (DMEM, Gibco Inc, Carlsbad, CA) supplemented with streptomycin 100 μg/mL, penicillin sulfate 100 U/mL, and 10% heat-inactivated fetal calf serum (Gibco Inc). The cytotoxicity of generation 3 PAMAM and generation 4 PPI dendrimers on both cells were evaluated using a 3-(4,5-dimethythiazol-2-yl)-2,5-diphenyl tetrazolium bromide (MTT) assay, which is the most frequently used method for measuring cell proliferation and viability. MCF-7 or A549 cells were seeded in 96-well culture plates for 48 hours at a density of 104 cells per well before the cytotoxicity assay. After that, the cells were treated with DMEM containing different concentrations of PAMAM or PPI dendrimers (dendrimer concentration ranges from 3.62 × 10−7 M to 7.25 × 10−5 M) for 48 hours, followed by removal of the medium, washing of the MCF-7 or A549 cells twice with fresh phosphate-buffered saline, and incubation of the cells with DMEM containing MTT for 3 hours. The MTT dye was reduced to purple formazan in living cells and the formazan generated was dissolved by dimethylsulfoxide. Absorbance of the solution in each well was measured at 570 nm using a microplate reader (MQX200R, Bio-Tek Instruments Inc, Winooski, VT). The cells that received no dendrimer were set as positive controls to 100% viability. The viabilities of the PAMAM and PPI dendrimers were expressed as a percentage of the control. Six repeats were conducted for each sample.
Acridine orange and ethidium bromide were used for morphological detection of apoptotic and necrotic cells.Citation26 MCF-7 and A549 cells were seeded in 24-well plates and cultured in the absence or presence of dendrimers (14.5 μM PAMAM or PPI dendrimer in DMEM) for 12 hours before the acridine orange/ethidium bromide double staining experiment. The cells were rinsed in phosphate-buffered saline twice and incubated in acridine orange/ethidium bromide containing phosphate-buffered saline (5 μg/mL acridine orange and 5 μg/mL ethidium bromide) at 37°C in 5% CO2 for 10 minutes. The stained cells were then imaged using a fluorescence microscope (Moticam 5000, Motic Instruments Inc, Richmond, Canada).
Results and discussion
Drug loading ability of PAMAM and PPI dendrimers
Phenylbutazone is a nonsteroidal anti-inflammatory drug for the long-term treatment of chronic pain particularly due to osteoarthritis and rheumatoid arthritis. It has an extremely low solubility of around 74 μM in distilled water, indicating low bioavailability by oral administration route and challenges in preparation of injections. Here phenylbutazone is used as a model drug to evaluate the drug-loading ability of PAMAM and PPI dendrimers. As shown in , the solubility of phenylbutazone was enhanced by a factor of 126 in the presence of 0.36 mM generation 3 PAMAM dendrimer, suggesting that an average number of 26 drug molecules were bound by each dendrimer. The solubility of phenylbutazone in PAMAM dendrimer solution is linear with dendrimer concentration due to increasing surface amine groups and interior pockets. Surprisingly, PPI dendrimer at a concentration of 0.36 mM only enhances phenylbutazone solubility by 13 times, and the solubilization behavior scarcely increases with dendrimer concentration. Our previous studies have demonstrated that surface amine groups play important roles in drug binding and the surface ionic interactions contribute much more to the solubility enhancement of drugs than interior encapsulations by hydrophobic and hydrogen bond interactions.Citation13 Here, both generation 4 PPI and generation 3 PAMAM dendrimers have 32 primary amine groups on their surface. The pKa values for these surface amine groups are 9.75Citation17 and 10.5Citation27 for PPI and PAMAM dendrimers, respectively, and the pH value of the dendrimer-drug solution is in the range of 5.3–6.0 for PPI and 6.3–7.0 for PAMAM depending on the ratio of dendrimer and drug in the solution, suggesting that all the amine groups are protonated and the numbers of charged surface functionalities for both dendrimers are equal to each other. Therefore, the distinct solubilization behaviors of PAMAM and PPI dendrimers cannot be interpreted by the number of surface charges.
Figure 1 Solubility enhancement of phenylbutazone in the presence of generation 3 PAMAM and generation 4 PPI dendrimers.
Abbreviations: PAMAM, polyamidoamine; PPI, polypropylenimine.
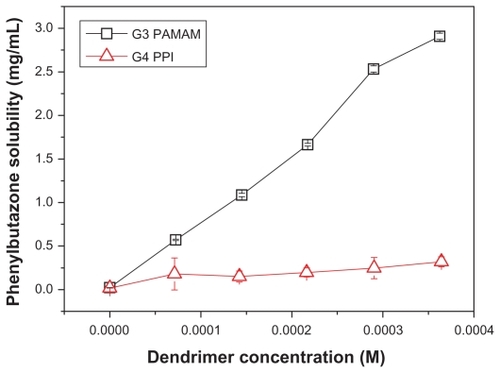
Interior hydrophobicity, pocket volume, amido groups, and surface charge densities should be considered in addition to surface charge. With regard to interior hydrophobicity, generation 4 PPI has a much more hydrophobic interior than generation 3 PAMAM, suggesting that PPI should be able to encapsulate more drugs in its interior pockets than PAMAM,Citation16,Citation28 which is opposite to the results shown in . For pocket volume, it is known that the length of branching units for constructing PPI dendrimer is much shorter than that for PAMAM dendrimer (4-bond versus 7-bond), thereby the size of generation 4 PPI is much smaller than that of generation 3 PAMAM (molecular weight 3513 Da versus 6900 Da; size 2.3 nmCitation29 versus 3.0 nmCitation1). A larger dendrimer size or pocket volume of PAMAM is likely to encapsulate more drug molecules. Further, the amido groups in the scaffold of PAMAM (60 amido groups in each generation 3 PAMAM) may facilitate the encapsulation of phenylbutazone molecules via hydrogen bond interactions (N-H in the amido group as hydrogen bond donor and oxygen atoms in phenylbutazone as receptors). Citation21 However, the effects of pocket volume and amido groups should be ruled out because more phenylbutazone molecules were found in the cavities of PPI dendrimer, which is evident by NOESY analysis and will be further discussed in the next sections. Finally, with regard to surface charge density, PPI with a smaller molecular size had a higher surface charge density than PAMAM (19.3 × 10−3/Å2 versus 11.3 × 10−3/Å2). Higher charge density on the globular surface of PPI dendrimer means steric hindrance during ionic binding which may decrease the amount of drug bound on the dendrimer surface. However, surface charge density was found to have a limited influence on the drug-loading ability of the dendrimer in our previous studies and cannot be a predominant factor in the distinct solubilization behaviors of PAMAM and PPI dendrimers towards phenylbutazone.Citation25,Citation30
A reasonable interpretation is that PPI and phenylbutazone complex is not stable in aqueous solution and may precipitate from the solution. This is confirmed by the disappearance of PPI dendrimer peaks in the 1H NMR spectra of the supernatant of PPI-phenylbutazone mixtures from aqueous solubility studies (data not shown). A possible reason for the precipitation of PPI-phenylbutazone complexes is that the PPI dendrimer is an amphiphilic macromolecule with a hydrophobic interior.Citation17 Attachment of phenylbutazone molecules on the PPI surface via ionic interaction neutralizes the surface charges of the dendrimer, forming hydrophobic nanostructures with low aqueous solubility. Another reason is that phenylbutazone encapsulated in the congested pockets of PPI dendrimer may interact with the alkyl chain of PPI by strong hydrophobic interactions, resulting in collapse of the dendritic scaffold into hydrophobic pellets and precipitation of the PPI-phenylbutazone complex from the solution.Citation22 Perhaps a combined effect of the two factors contributes to precipitation of the complex. In the case of the PAMAM dendrimer, its interior cavities are much larger than those of PPI and the microenvironment of PAMAM is relatively hydrophilic, which make drug complexes with PAMAM more soluble than those with PPI in aqueous solutions. Precipitation of PPI-phenylbutazone complexes and stability of the PAMAM-phenylbutazone complex were further confirmed by a gradient-feed method.
Drug-loading ability of PAMAM and PPI dendrimers
Using the gradient-feed method, equivalent amounts of phenylbutazone were added into the dendrimer solutions in a stepwise manner rather than single addition of excess drugs in the phase solubility test. As shown in , a similar amount of phenylbutazone was bound by PAMAM and PPI dendrimers at the initial steps of the gradient-feed experiment. The drugs solubilized by both dendrimers increased linearly with the total amount of added drugs at this stage, which is attributed to the equivalent number of surface charges and interior pockets for generation 3 PAMAM and generation 4 PPI dendrimer. A maximum amount of phenylbutazone (2.4 mg) solubilized by PPI dendrimer was obtained at the ninth step when a cumulative amount of 3.6 mg phenylbutazone was fed, and a remarkable decrease in the amount of solubilized drug by PPI was observed in the following steps. The drug concentration decreased to 0.11 mg/mL which is slightly higher than the solubility of phenylbutazone in distilled water at the twentieth step, and white particles were found on the walls of the microtest tubes, suggesting precipitation of PPI-drug complexes during this period. As discussed above, precipitation of PPI and phenylbutazone complexes is caused by neutralization of surface charges on the surface of PPI dendrimer with a hydrophobic interior and/or the collapse of PPI scaffolds by strong hydrophobic interactions. The phenylbutazone amount of 2.4 mg can be recognized as the maximum drug-loading capacity for 0.14 mM generation 4 PPI dendrimer in 4 mL water. In the case of the PAMAM dendrimer, the amount of phenylbutazone dissolved in dendrimer solution linearly increases from 0.28 to 3.6 mg in the first 13 steps, followed by a slight increase in drug concentration, and the drug does not reach its saturation point in PAMAM solution even at the twentieth step. Therefore, the PAMAM-phenylbutazone complex is much more stable than the PPI-drug complex in aqueous solution, which explains the difference in solubilization behavior between the PAMAM and the PPI dendrimer in the phase solubility studies. It is concluded that the results obtained from phase solubility studies cannot fully clarify the loading ability of the dendrimer, given that the PPI dendrimer failed to enhance the solubility of phenylbutazone greatly, but exhibited high drug-loading efficiency before a maximum loading amount was achieved in the gradient-feed experiment.
Characterization of PAMAM-phenylbutazone and PPI-phenylbutazone
To clarify the structural differences between PAMAM and PPI dendrimers and their influence on drug-loading, 1H NMR and NOESY spectra of the dendrimers and their complexes with phenylbutazone were obtained. As shown in , generation 4 PPI dendrimer has four groups of peaks in D2O which are assigned as protons HA, HB′, HB′ and HC, respectively, while generation 3 PAMAM dendrimers has six groups of peaks which are assigned as Ha, Hb, Hb′, Hc, Hd, and Hd′, respectively.Citation3,Citation18 Protons (Hc) of PPI and protons (Hb′ and Hd′) of PAMAM exhibit significant downfield shifts in dendrimer-phenylbutazone complexes as compared with that in free dendrimers. In addition, significant upfield shifts for other protons of PPI and PAMAM dendrimers are observed in the complexes. Previous studies have demonstrated that downfield shift of the methylene protons located on the surface of amine-terminated PAMAM dendrimer is attributed to the ionic binding of guests on dendrimer surface (decreased electron density around these protons) and upfield shift of the interior methylene protons in PAMAM pockets might be caused by the hydrophobic encapsulations of the guests (increased electron density around these protons).Citation21 The shift behaviors of protons in PAMAM and PPI dendrimers in the presence of phenylbutazone prove the roles of ionic interactions and interior encapsulations in the formation of dendrimer-phenylbutazone complexes.
Figure 3 1H Nuclear magnetic resonance spectra of dendrimer and dendrimer-phenylbutazone complexes. (A) generation 4 PPI, (B) generation 4 PPI-phenylbutazone complex, (C) generation 3 PAMAM, and (D) generation 3 PAMAM-phenylbutazone complex.
Abbreviations: PAMAM, polyamidoamine; PPI, polypropylenimine.
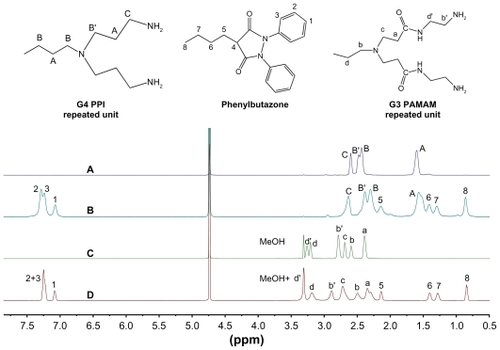
The encapsulations of phenylbutazone molecules in generation 3 PAMAM and generation 4 PPI dendrimers are investigated by a 1H-1H NOESY study, which is always used to analyze the spatial distance between specific protons.Citation21 The presence of NOE cross-peaks between two protons in NOESY spectrum indicates the spatial proximity of these protons, and the cross-peak intensity decreases with spatial distance, and increases with the number of molecules involved in the cross-peak.Citation3,Citation31 As shown in , strong cross-peaks between aromatic protons (H1–3) and the interior methylene protons (HA and HB) are observed, confirming that phenylbutazone molecules are encapsulated in the pockets of PPI dendrimer. The absence of cross-peaks between the protons (HB′ and HC) of PPI and phenylbutazone suggests that most of the drugs are located deep inside the interior of PPI, and this phenomenon is in accordance with our previous findings on PAMAM-drug complexes as well as the NOESY results for PAMAM-phenylbutazone complexes shown in .Citation21 The negative NOE cross-peaks between the aromatic protons (H1–3) and the alkyl protons (H5–8) of phenylbutazone further demonstrate the bound of drug molecules by PPI dendrimer.Citation3,Citation25 In case of PAMAM-phenylbutazone complex, cross-peaks between PAMAM and phenylbutazone are found between Ha–d of PAMAM and H2,3 of phenylbutazone. The absence of cross-peaks between H1 and Ha–d and the absence of cross-peaks between H1 and H5–8 indicate that the drugs and dendritic scaffold are more distant from each other than that in PPI-phenylbutazone complex. This is attributed to the fact that PPI has a more hydrophobic interior than PAMAM. The 1H NMR and NOESY analysis concluded that both ionic interaction and interior encapsulation play important roles in the formations dendrimer-phenylbutazone complexes. The neutralization of surface charges on PPI surface via ionic interactions and the collapse of PPI structure due to strong hydrophobic interactions may lead to the precipitation of PPI-phenylbutazone complexes which is confirmed in a previous section.
Release behavior of phenylbutazone from PAMAM and PPI complexes
To reveal further the structure differences in PAMAM and PPI dendrimer, in vitro release behaviors of phenylbutazone from PAMAM and PPI matrixes were investigated. As shown in , generation 3 PAMAM dendrimer shows better sustained release ability than generation 4 PPI dendrimer, ie, only 13% of the drug was found in outer phase of the dialysis bag after 12 hours for PAMAM, while 44% of the drug released from PPI-drug complex during the same period. In phase solubility and gradient-feed experiments, the generation 3 PAMAM dendrimer shows better capacity in loading phenylbutazone molecules compared with the generation 4 PPI dendrimer at high phenylbutazone/dendrimer feeding ratios, and the generation 3 PAMAM-phenylbutazone complex is much more stable in aqueous solution than in the generation 4 PPI-phenylbutazone complex. Therefore, a faster release of phenylbutazone was obtained from PPI than from PAMAM. Drug carriers with ideal sustained release behavior can improve drug bioavailability, decrease side effects of a drug at a high concentration, simplify the dosing schedule, and are beneficial for practical applications.Citation32 A PAMAM dendrimer which exhibits a much slower release rate for phenylbutazone should be a better candidate in the development of dendrimer- phenylbutazone formulations than PPI dendrimer. Perhaps chemical modification of PPI surface with hydrophilic moieties such as PEG chains and acetyl groups may increase the stability of the dendrimer-drug complex, improve solubility and delivery efficacy of the PPI dendrimer,Citation33 and solve the serious toxicity problem of the PPI dendrimer which will be discussed in the next section.
Comparison of cytotoxicity on PAMAM and PPI dendrimers
An MTT assay was conducted to compare the cytotoxicity of PAMAM and PPI dendrimers. shows the toxicity of PAMAM and PPI dendrimers in A549 cells. Generation 3 PAMAM is not toxic at concentrations up to 72 μM, while generation 4 PPI exhibits cytotoxicity at a concentration of 1.4 μM, suggesting that generation 4 PPI is at least 50 times more toxic to A549 cells than generation 3 PAMAM. Similar results were obtained for MCF-7 cells as shown in . The IC50 values of generation 4 PPI dendrimer in A549 and MCF-7 cells are 4.3 μM and 4.1 μM, respectively. An acridine orange/ethidium bromide double staining method was also used to compare the cytotoxicity of PAMAM and PPI dendrimers.Citation26 As shown in , A549 and MCF-7 cells incubated with generation 3 PAMAM are viable (green), but cells treated with generation 4 PPI dendrimer at an equivalent molar concentration are found at a lower cell density and cells in necrosis with orange nuclei. Previous studies have found that primary amine groups on the dendrimer surface interact with phospholipids in the cell membrane,Citation33 followed by disturbance of these amphiphilic molecules and formation of holes in the cell membrane, resulting in leakage of intracellular components.Citation34 Cationic dendrimers were reported to destroy mitochondrial membranes, leading to the generation of reactive oxygen species causing oxidative stress, DNA damage, and apoptosis.Citation35,Citation36 The removal of surface amine groups by acetylation or PEGylation can effectively decrease the cytotoxicity of PAMAM and PPI dendrimers,Citation23,Citation37 indicating that surface charge plays an important role in the cytotoxicity of both dendrimers.Citation34 Generation 3 PAMAM and generation 4 PPI have equivalent numbers of primary amine groups (pKa about 10) which are fully protonated under physiological conditions (pH about 7.4). Also, they have a similar pH-buffering capacity due to the same amount of tertiary amine groups, indicating similar escape abilities for PAMAM and PPI from endosomes after cellular uptake.Citation37 Therefore, other factors including dendrimer size and interior hydrophobicity, are proposed to explain the distinct viability of cells in the presence of generation 3 PAMAM and generation 4 PPI. Dendrimer cytotoxicity was found to be proportional to dendrimer size or generation in many cell lines,Citation34 ie, generation 4 and 5 PAMAM dendrimers show much higher cytotoxicity to MCF-7 cells than generation 3 PAMAM dendrimers at an equivalent concentration of surface charge (). Also, high-generation PPI dendrimer displayed higher cytotoxicity than low-generation PPI dendrimer on many cell lines.Citation34,Citation38 In that situation, generation 4 PPI should be more biocompatible than generation 3 PAMAM with a larger size and equivalent amount of surface charge. Perhaps the unique hydrophobic interior of PPI plays an important role in the serious toxicity of the PPI dendrimer. Detailed mechanisms for this interesting phenomenon are still under investigation.
Figure 6 Viability of A549 cells (A) and MCF-7 cells (B) incubated with different concentrations of generation 3 PAMAM and generation 4 PPI dendrimers for 48 hours.
Figure 7 Images of A549 (A and B) and MCF-7 cells (C and D) incubated with generation 3 PAMAM (A and C), or generation 4 PPI (B and D) for 12 hours at a concentration of 14.5 μM.
Figure 8 Viability of MCF-7 cells incubated with generation 3, generation 4, and generation 5 PAMAM dendrimers at different concentrations for 48 hours.
Abbreviation: PAMAM, polyamidoamine.
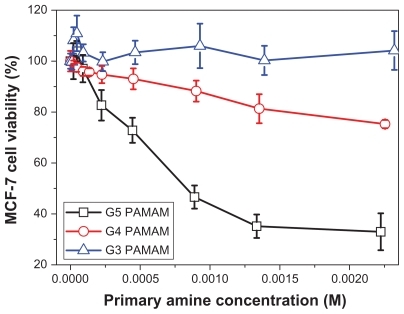
Scheme 1 Molecular structures of PAMAM (A) and PPI (B) dendrimers.
Abbreviations: PAMAM, polyamidoamine; PPI, polypropylenimine.
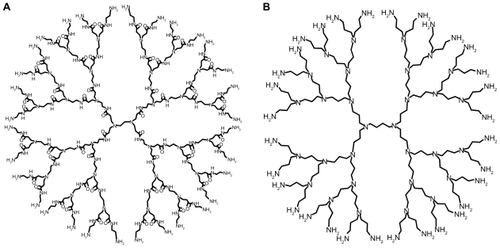
Abbreviations: PAMAM, polyamidoamine; PPI, polypropylenimine.
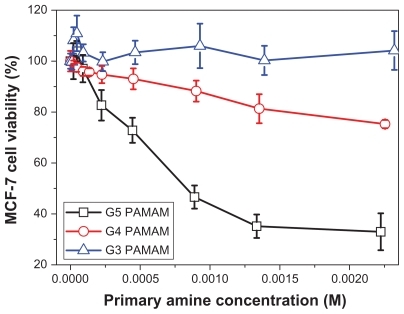
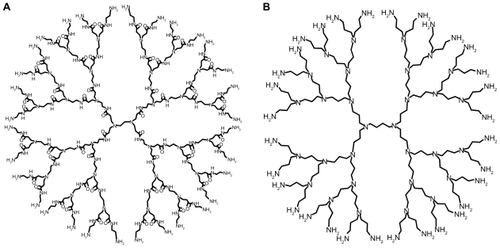

Abbreviations: PAMAM, polyamidoamine; PPI, polypropylenimine.
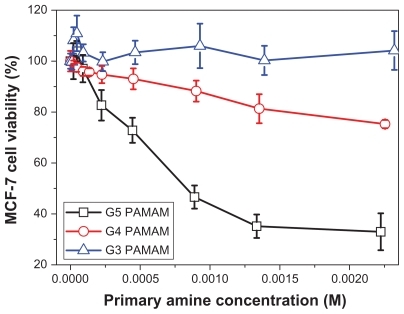
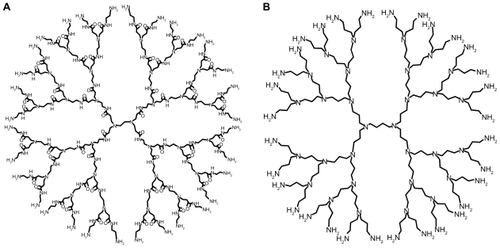

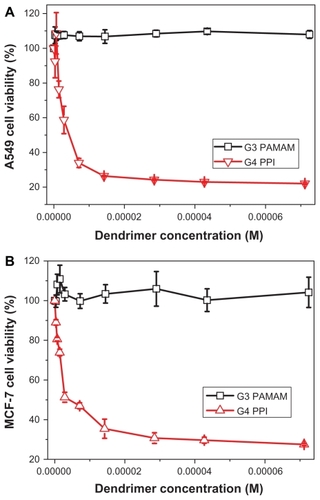
Conclusion
In the present study, we compared the drug loading ability, release behavior, and cytotoxicity of generation 3 PAMAM and generation 4 PPI dendrimers. As shown in , generation 3 PAMAM showed better performance in solubilization and release of phenylbutazone molecules than did generation 4 PPI with the same number of surface amine groups. Also, generation 4 PPI dendrimer was at least 50 times more toxic than generation 3 PAMAM dendrimer to MCF-7 and A549 cells. The generation 4 PPI-phenylbutazone complex is not as stable as the generation 3 PAMAM-phenylbutazone complex and precipitates from aqueous solution above the maximum loading capacity. NMR studies confirmed strong hydrophobic interactions between phenylbutazone molecules and dendritic scaffolds in the interior pockets of the generation 4 PPI dendrimer, which is proposed to be the major reason behind precipitation of the generation 4 PPI-phenylbutazone complex. In conclusion, generation 3 PAMAM dendrimers are better candidates for development of a phenylbutazone formulation, while generation 4 PPI dendrimers should be surface-modified to improve their performance in terms of drug-loading and release and to solve their toxicity problems before being used as drug carriers.Citation39
Table 1 A systematic comparison of G3 PAMAM and G4 PPI dendrimers on their physicochemical properties, drug loading, stability, complex structure, release rate, and cytotoxicity
Disclosure
The authors report no conflicts of interest in this work.
Acknowledgments
We thank the Talent Program of East China Normal University (77202201), the “Dawn” Program of Shanghai Education Commission (10SG27), and the 2010 Open Foundation of the CAS Key Laboratory for Biomedical Effects of Nanomaterials and Nanosafety for supporting this research.
References
- TomaliaDABirth of a new macromolecular architecture: dendrimers as quantized building blocks for nanoscale synthetic polymer chemistryProg Polym Sci200530294324
- MenjogeARKannanRMTomaliaDADendrimer-based drug and imaging conjugates: design considerations for nanomedical applicationsDrug Discov Today20101517118520116448
- ZhaoLBWuQLChengYYZhangJHWuJHXuTWHigh- throughput screening of dendrimer-binding drugsJ Am Chem Soc2010132131821318420825185
- AstrucDBoisselierEOrnelasCDendrimers designed for functions: from physical, photophysical, and supramolecular properties to applications in sensing, catalysis, molecular electronics, photonics, and nanomedicineChem Rev20101101857185920356105
- ChengYYXuTWThe effect of dendrimers on the pharmacodynamic and pharmacokinetic behaviors of non-covalently or covalently attached drugsEur J Med Chem2008432291229718276038
- GilliesERFrechetJMJDendrimers and dendritic polymers in drug deliveryDrug Discov Today200510354315676297
- ZhangYQSunYHXuXPSynthesis, biodistribution, and microsingle photon emission computed tomography (SPECT) imaging study of technetium-99m labeled PEGylated dendrimer poly(amidoamine) (PAMAM)-folic acid conjugatesJ Med Chem2010533262327220350006
- ZhangYQSunYHXuXPRadiosynthesis and micro-SPECT imaging of 99m Tc-dendrimer poly(amido)-amine folic acid conjugateBioorg Med Chem Lett20102092793120045643
- XuXPZhangYQWangXRadiosynthesis, biodistribution and micro-SPECT imaging study of dendrimer-avidin conjugateBioorg Med Chem2011191643164821310621
- MintzerMAGrinstaffMWBiomedical applications of dendrimers: a tutorialChem Soc Rev20114017319020877875
- TomaliaDABakerHDewaldJA new class of polymers: starburst-dendritic macromoleculesPolym J198517117132
- de Brabander van den BergEMMMeijerEWPoly(propylene imine) dendrimers: large-scale synthesis by heterogeneously catalyzed hydrogenationsAngew Chem Int Ed19933213081311
- ChengYYWuQLLiYWXuTWExternal electrostatic interaction versus internal encapsulation between cationic dendrimers and negatively charged drugs: which contributes more to solubility enhancement of the drugsJ Phys Chem B20081128884889018605754
- NaylorAMGoddardWAIIIKieferGETomaliaDAStarburst dendrimers. 5. molecular shape controlJ Am Chem Soc198911123392341
- ChengYYLiYWWuQLXuTWNew insights into the interactions between dendrimers and surfactants by two dimensional NOE NMR spectroscopyJ Phys Chem B2008112126741268018783265
- Richter-EggerDLTesfaiATuckerSASpectroscopic investigations of poly(Propyleneimine) dendrimers using the solvatochromic probe phenol blue and comparisons to poly(amidoamine) dendrimersAnal Chem2001735743575111774916
- KannaiyanDImaeTpH-dependent encapsulation of pyrene in PPI-core: PAMAM-shell dendrimersLangmuir2009255282528519397362
- BoisselierELiangLDalko-CsibaMRuizJAstrucDInteractions and encapsulations vitamins C, B3, and B6 with dendrimer in waterChem Eur J2010166056606820397156
- HabaYHaradaATakagishiTKonoKRendering poly(amidoamine) or poly(propylenimine) dendrimers temperature sensitiveJ Am Chem Soc2004126127601276115469255
- ChengYYWangJRRaoTLHeXXXuTWPharmaceutical applications of dendrimers: promising nanocarriers for drug deliveryFront Biosci2008131447147117981642
- HuJJChengYYMaYRWuQLXuTWHost-guest chemistry and physico-chemical properties of dendrimer-mycophenolic acid complexesJ Phys Chem B2009113647419063655
- YangKChengYYFengXYZhangJHWuQLXuTWInsights into the interactions between dendrimers and multiple surfactants: 6. Formation of miscellaneous mixed micelles revealed by a combination of 1H NMR, diffusion, and NOE analysisJ Phys Chem B20101147265727320462223
- YangKWengLChengYYHost-guest chemistry of dendrimer- drug complexes. 6. fully acetylated dendrimers as biocompatible drug vehicles using dexamethasone 21-phosphate as a model drugJ Phys Chem B20111152185219521338144
- ChengYYXuZHMaMLXuTWDendrimers as drug carriers: applications in different routes of drug administrationJ Pharm Sci20089712314317721949
- YangWJLiYWChengYYWuQLWenLPXuTWEvaluation of phenylbutazone and poly(amidoamine) dendrimers interactions by a combination of solubility, 2D-NOESY NMR, and isothermal titration calorimetry studiesJ Pharm Sci2009981075108518680167
- LiuJYPangYHuangWZhuXYZhouYFYanDYSelf-assembly of phospholipid- analogous hyperbranched polymers nanomicelles for drug deliveryBiomaterials2010311334134119883937
- D’EmanueleAAttwoodDDendrimer-drug interactionsAdv Drug Deliv Rev2005572147216216310283
- KojimaCToiYHaradaAKonoKPreparation of poly(ethylene glycol)-attached dendrimers encapsulating photosensitizers for application to photodynamic therapyBioconjug Chem20071866367017375896
- VohsJKBregeJJRaymondJEBrownAEWilliamsGLFahlmanBDLow-temperature growth of carbon nanotubes from the catalytic decomposition of carbon tetrachlorideJ Am Chem Soc20041269936993715303864
- ChengYYLiYWWuQLZhangJHXuTWGeneration-dependent encapsulation/electrostatic attachment of phenobarbital molecules by poly(amidoamine) dendrimers: evidence from 2D-NOESY investigationsEur J Med Chem2009442219222318635290
- ZhaoLBChengYYHuJJWuQLXuTWHost-guest chemistry of dendrimer-drug complexes. 3. Competitive binding of multiple drugs by a single dendrimer for combination therapyJ Phys Chem B2009113141721417919788172
- MedinaSHEI-SayedMEDendrimers as carriers for delivery of chemotherapeutic agentsChem Rev20091093141315719534493
- ChengYYZhaoLBLiYWXuTWDesign of biocompatible dendrimers for cancer diagnosis and therapy: current status and future perspectivesChem Soc Rev2011402673270321286593
- DuncanRIzzoLDendrimer biocompatibility and toxicityAdv Drug Deliv Rev2005572215223716297497
- KuoJSJanMSLinYInteractions between U-937 human macrophages and poly(propyleneimine) dendrimersJ Control Release2007120515917537537
- ThomasTPMajorosIJKotlyarAMullenDBanaszak HollMMBakerJRJrCationic poly(amidoamine) dendrimer induces lysosomal apoptotic pathway at therapeutically relevant concentrationsBiomacromolecules2009103207321419924846
- WaiteCLSparksSMUhrichKERothCMAcetylation of PAMAM dendrimers for cellular delivery of siRNABMC Biotechnol200993819389227
- JainKKesharwaniPGuptaUJainNKDendrimer toxicity: Let’s meet the challengeInt J Pharm201039412214220433913
- KolhatkarRBKitchensKMSwaanPWGhandehariHSurface acetylation of polyamidoamine (PAMAM) dendrimers decreases cytotoxicity while maintaining membrane permeabilityBioconjug Chem2007182054206017960872