Abstract
Biomaterials and biomedical devices induced life-threatening bacterial infections and other biological adverse effects such as thrombosis and fibrosis have posed a significant threat to global healthcare. Bacterial infections and adverse biological effects are often caused by the formation of microbial biofilms and the adherence of various biomacromolecules, such as platelets, proteins, fibroblasts, and immune cells, to the surfaces of biomaterials and biomedical devices. Due to the programmed interconnected networking of bacteria in microbial biofilms, they are challenging to treat and can withstand several doses of antibiotics. Additionally, antibiotics can kill bacteria but do not prevent the adsorption of biomacromolecules from physiological fluids or implanting sites, which generates a conditioning layer that promotes bacteria’s reattachment, development, and eventual biofilm formation. In these viewpoints, we highlighted the magnitude of biomaterials and biomedical device-induced infections, the role of biofilm formation, and biomacromolecule adhesion in human pathogenesis. We then discussed the solutions practiced in healthcare systems for curing biomaterials and biomedical device-induced infections and their limitations. Moreover, this review comprehensively elaborated on the recent advances in designing and fabricating biomaterials and biomedical devices with these three properties: antibacterial (bacterial killing), antibiofilm (biofilm inhibition/prevention), and antibiofouling (biofouling inhibition/prevention) against microbial species and against the adhesion of other biomacromolecules. Besides we also recommended potential directions for further investigations.
Introduction
Numerous studies have been conducted in order to develop and fabricate antibacterial and antibiofouling biomaterials and biomedical devices. Irrespective of this, the quest to develop biomaterials and biomedical devices with excellent antibacterial and antibiofouling properties is still in progress since pathogenic bacterial strains are developing resistance to various antimicrobial treatments.Citation1,Citation2 Bacterial contamination of biomaterials and biomedical devices not only compromises their efficacy but is also a leading cause of bacterial infections.Citation3 According to a study reported by Magill et al, about 26% of healthcare infections in the United States alone are caused due to the microbial contamination of these biomaterials and biomedical devices,Citation4 and further, each year in the United States, 1.7 million individuals suffer from healthcare-associated infections, with about 90,000 dying as a result of these infections.Citation2,Citation5 These statistics exclude healthcare-associated infections that occur in intensive care units, where this patient population makes up only a fraction of the entire population, but the probability of infection-related mortality is substantially higher owing to the patient’s compromised health status. For example, about 80,000 bloodstream infections associated with central venous catheters occur annually in intensive care units in the United States, with a mortality rate of 12–25%.Citation1,Citation6 The estimated annual medical costs of these patients’ issues are between $296 million and $2.3 billion.Citation6,Citation7
Biomaterials and biomedical devices, including contact lenses, drug delivery devices, catheters, protective apparel, prosthetic devices, sensors, surgical equipment, pacemaker, and lens cases, are often contaminated by pathogenic microorganisms through biofilm formation.Citation8–10 In 1982, an electron microscopy examination of a pacemaker lead in a patient with recurrent Staphylococcus aureus bloodstream infection gave the first evidence of biofilm involvement in device-related infections ().Citation11, Since then, almost all types of indwelling biomaterials and biomedical devices have been associated with the occurrence of microbial biofilms.Citation12,Citation13 Following decades of research, it has been consistently demonstrated that biofilms play a vital role in the pathogenesis of tissue-associated infections (). Moreover, it was found that the extensive use of a variety of indwelling biomaterials and biomedical devices implanted in humans potentially promotes microbial adhesion and colonization, ultimately leading to infection.Citation12
Figure 1 Pathogenic microbial biofilms contaminated biomaterials and biomedical devices induced infections.
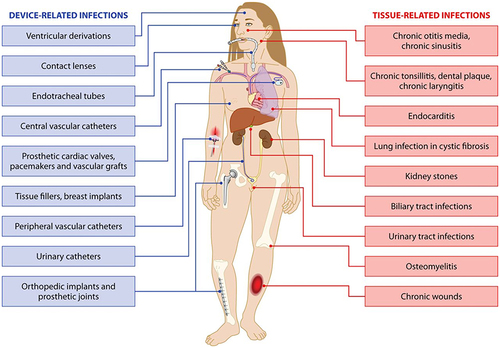
Biofilm Formation on Biomaterials and Biomedical Devices
The pathogenic bacterial strains form biofilms by adhering to the surfaces of biomaterials and biomedical devices. Initially, bacterial adhesion to the abiotic surfaces of biomaterials and biomedical devices is typically non-specific, resulting from a variety of non-specific forces [electrostatic interactions (acid-base interactions), Van der Waals interactions, etc], and this type of adhesion is called passive adhesion (). Bacterial adhesion is accomplished by their interactions with the adhesins of biomaterials and biomedical devices. During such interactions, pathogenic bacteria behave like colloidal particles.Citation15–19 Additionally, bacterial cell filamentous appendages (pili, nanofibers, flagella, etc), and species-specific proteins (autolysins) also act as adhesins, promoting their attachment to the abiotic surfaces.Citation15,Citation19–24
Figure 2 Staphylococcus aureus adherence to the surface of biomaterials and biomedical devices.
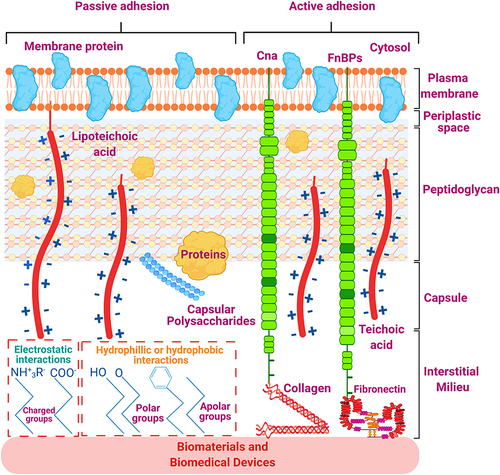
On the other hand, when biomaterials and biomedical devices are immersed in the physiological fluid, their bare surfaces are rapidly covered by extracellular matrix proteins and immune protein components, leading to the generation of a biotic coating (conditioning layer).Citation25,Citation26 Thus, bacterial adhesion to the biotically coated surfaces of biomaterials and biomedical devices is usually specific adhesin-based interactions, commonly known as active adhesion accompanied by irreversible active mechanisms.Citation19,Citation27 The active adhesion is often mediated by the biotic coating of three extracellular matrix proteins (fibrinogen, fibronectin, and collagen), which serve as ligands for bacterial attachment to biomaterials and biomedical devices ().Citation15,Citation19,Citation28
After attachment, bacterial species stick to one another and form numerous colonies.Citation8,Citation29 The adherent bacterial colonies are further embedded in biomaterials and biomedical devices by continuously producing an extracellular matrix composed of extracellular polymer substances.Citation15 The extracellular polymer substances are composed of extracellular DNA, lipids, proteins, and polysaccharides (). The extracellular polymer substances play a vital role in forming and sustaining microbial biofilms. For example, polysaccharide intracellular adhesin in S. epidermidis and S. aureus promote their adhesion to the surfaces of biomaterials and biomedical devices.Citation15
Figure 3 Transitions in the development of a biofilm.
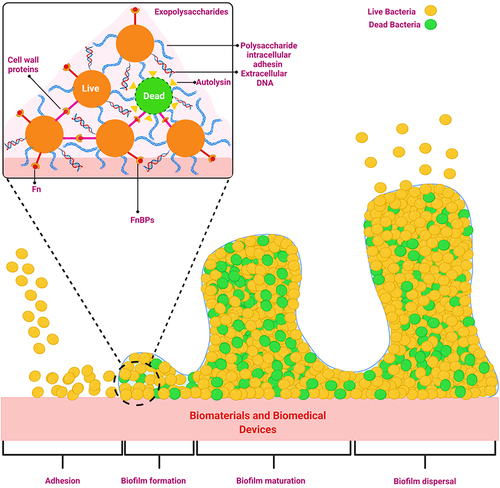
Moreover, polysaccharide intracellular adhesin-positive (presence of cell-surface components or appendages) bacterial species are more resistant to antibiotics compared to polysaccharide intracellular adhesin-negative (absence of cell-surface components or appendages) bacterial strains.Citation15,Citation30,Citation31 While extracellular DNA plays a significant role in nutrient supply, transferring the genes among cells, stabilizes, and strengthens the extracellular biofilm matrix.Citation15,Citation32–34 Due to such behaviour of pathogenic bacterial colonies in biofilms, they can resist several dozes of antibacterial compounds or drugs.Citation3,Citation9,Citation35–37
Biofouling of Biomaterials and Biomedical Devices
Biofouling is an additional important element that facilitates the formation of biofilms by pathogenic bacterial strains on biomaterials and biomedical devices.Citation1,Citation8,Citation38 Biofouling is typically medicated by the adsorption of live/dead planktonic bacterial cells, proteins (streptavidin, lysozyme, fibronectin, fibrinogen, bovine serum albumin, etc), and platelets on the surfaces of biomaterials and biomedical devices.Citation19 The initial adhesion in the case of microbial species is most probably caused by a small population of microbes that aggregate on the surface after biomaterial or device implantation, either via contact with the skin flora of the patient/medical professional or through microbial diffusion from the implant site.Citation1 These adsorbed live/dead bacterial cells further act as a platform for attaching the microbial cells and their proliferation into biofilms on the surfaces of biomaterials and biomedical devices.Citation8,Citation29 The most straightforward methods have focused on preventing microbial adherence (antibiofilm) or eradicating adherent microorganisms (antimicrobial).
However, the conditioning film’s function is often ignored in both methods.Citation1 Upon the implantation of the biomedical device or biomaterials, the surface is readily coated with the available extracellular matrix, immune system proteins, blood biomacromolecules (proteins, etc), and platelets referred to as the biotic coating or conditioning film ().Citation1,Citation39,Citation40 The surface chemistry and wettability of biomaterials and biomedical devices are critical for the adhesion of various adhesins to their surfaces.Citation15,Citation41 Although biomaterials or biomedical devices are initially unfavorable for microbial adherence, the formation of conditioning films eventually results in microbial adhesion and subsequent growth into biofilms ().Citation1 There is sufficient evidence supporting antibiofilm agents and technology’s in vitro biofilm prevention capabilities. Unfortunately, the majority of existing in vitro test methodologies do not include in vivo biomaterials and biomedical device conditions.Citation42 Therefore, scientists designing biomaterials and biomedical devices must consider the microenvironment of the implanting site and be required to investigate for non-specific adsorption of hosting biomacromolecules.Citation1 For example, the conditioning film in vascular catheters is generally composed of plasma proteins (fibrinogen, fibronectin, and albumin) and platelets (). In contrast, the conditioning film in urinary catheters is frequently composed of electrolytes and proteins from the patient’s urine.Citation1,Citation39,Citation43,Citation44
Figure 4 Biofilm formation on the biomedical device (catheter) mediated by conditioning film.
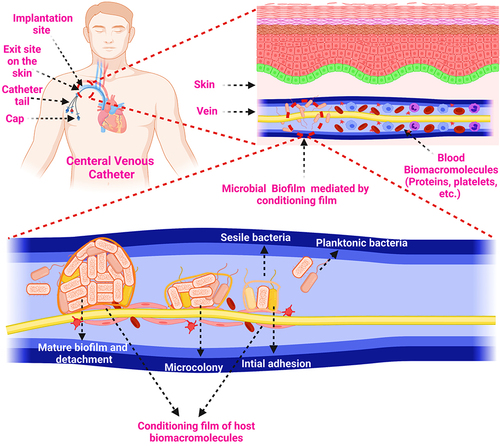
Figure 5 Schematic illustration of the biofouling of biomaterials and biomedical devices.
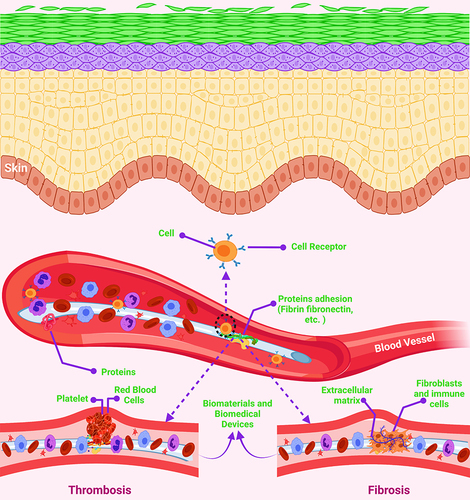
It has been demonstrated that bacteria responsible for infections caused by biomaterials and biomedical devices may readily attach to a variety of proteins through site-specific adhesion receptors and generate coagulase enzymes that promote microbial adhesion and thrombogenesis.Citation1,Citation47–49 Instead, substantial plasma protein adsorption by itself may promote platelet adhesion and activation, resulting in surface-induced thrombogenesis, which prevents blood flow through the blood-contacting devices, ultimately resulting in device failure and local tissue necrosis ().Citation1,Citation46,Citation50,Citation51 Furthermore, plasma protein adsorption also contributes to fibrosis-like adverse effects by providing the adhesion sites to fibroblast, immune cells, and extracellular matrix ().Citation46,Citation48
Solutions Practicing in Healthcare Systems for Curing the Biomaterials and Biomedical Devices-Induced Microbial Biofilms Infections and Their Limitations
Currently, many physicians employ oral or intravenous antibiotics before or soon after device implantation to decrease the incidence of infections caused by contaminated biomaterials and biomedical devices.Citation1,Citation52 Unintentionally, the extensive use of antibiotics has resulted in the development of many antibiotic-resistant bacteria, which have become a worldwide pandemic.Citation1,Citation53 The antibiotic drugs used for treating microbial biofilms-induced infections have poor efficacy against pathogenic bacteria infecting peri-implant tissues and require additional antibiotic treatment posing a significant risk of acquiring resistance.Citation1,Citation54 Moreover, antibiotic therapy does not address the adhesion issues of biomacromolecules on the surfaces of biomaterials and biomedical devices from implanting sites, compromising its effectiveness, resulting in microbial biofilms and biological biofouling reinfections. In this case, replacing biomaterials and biomedical devices is the only feasible alternative for preventing mortality and morbidity associated with bacterial infection. This approach may sometimes be found to be inefficient, complicated, or very expensive.Citation8,Citation55
Therefore, scientists have developed antimicrobial biomaterials and biomedical devices by adopting different strategies, including the incorporation of various bactericidal compounds (releasable/non-releasable), microbe repelling/releasing materials, and the potential combination of two onto their surfaces.Citation56–64 While these strategies appear to be very effective in killing and reducing microbial adherence and consequent implant-induced microbial infections, their effectiveness against other biomacromolecule adhesion is often ignored. As previously explained, they also have a significant role in biomaterials and biomedical devices-induced biological biofouling infections.
Solutions Practicing in Healthcare Systems for Curing the Biomaterials and Biomedical Devices-Induced Biological Biofouling Infections and Their Limitations
The current most frequently used clinical standard of treatment for preventing the biomaterials and biomedical devices-induced thrombogenesis and subsequent thrombosis (biological biofouling infections) is systemic heparin injections, which prohibit the formation of fibrin by actuating the coagulation inhibitor antithrombin.Citation65,Citation66 Numerous clinical studies have shown that heparin reduces the risk of thrombogenesis and thrombosis by modulating the coagulation cascade response.Citation67,Citation68 Nonetheless, using soluble heparin has no impact on platelet aggregation or activation, a crucial step in the formation of thrombus.Citation69,Citation70 In addition, the administration of systemic heparin depletes the patient’s whole blood supply of its clotting ability, which may result in stern complications such as heparin-induced thrombocytopenia (), hypertriglyceridemia, and postoperative bleeding.Citation71–74 As a result of these severe complications, systemic heparin administration continues to be the most considerable incidence of clinical drug-related mortality in the United States.Citation75,Citation76 Additionally, it has been demonstrated that using heparin in solution promotes the formation of bacterial biofilms on the surface of blood-contacting biomaterials and biomedical devices, thus raising the risk of hospital-associated infections.Citation70,Citation77
Figure 6 Heparin-induced thrombocytopenia pathogenicity.
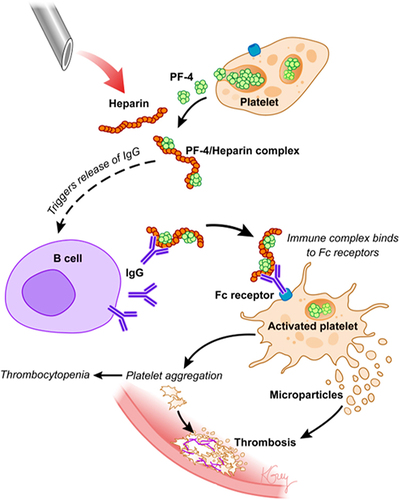
To obviate the deleterious consequences of systemic administration, the fabrication of localized heparin-based coatings has received considerable attention. Currently, there are two types of commercially available local heparin administration devices: heparin-eluting materials that release heparin from a polymeric matrix and surface-immobilized heparin materials.Citation66,Citation78 When compared to untreated controls, these heparinized materials showed superior clinical efficacy in terms of reducing thromboembolism and surgical complications.Citation79,Citation80 Additionally, immobilization of heparin prevents the formation of biofilms, which is due to the presence of negatively charged sulfates in heparin molecules, which inhibit bacteria’s adhesion to the surface.Citation81,Citation82 Regardless of these advantages and the commercial availability of heparinized materials, systemic heparin injections continue to be used.Citation70,Citation83,Citation84 The continued use of systemic heparin may be justified because it does not suppress platelet activation and, therefore, significantly contributes to thrombogenesis and eventual thrombosis (). While both thrombogenesis and thrombosis processes are ignored, large doses of injectable heparin are required to suppress the coagulation response before, during, and after implantation of heparin-coated biomaterials and biomedical devices; nevertheless, this over suppression is inadequate to avoid this thrombosis entirely.Citation70,Citation85
Effective Solutions for Preventing the Biomaterials and Biomedical Devices-Induced Microbial Biofilms and Biological Biofouling Infections
To be an effective solution for preventing biomaterials and biomedical devices-induced microbial biofilms and biological biofouling infections, they must exhibit not only superior growth-inhibitory efficacy against pathogenic bacterial species but also the ability to prevent the adhesion of live/dead microbiological species and non-specific/specific adhesion of proteins, platelets, and other biological biomacromolecules. Therefore, biomaterials and biomedical devices must be designed and fabricated with such materials that possess in situ excellent antibacterial, antibiofilm, and antibiofouling performances. Each failure may result in the contamination of biomaterials and biomedical devices with a variety of pathogenic bacterial strains, as well as other undesirable biological consequences (thrombosis and fibrosis).
Numerous reviews have been published on the design of biomaterials and biomedical devices for antibacterial, antibiofilm, and antibiofouling properties against microbial species. In addition, plentiful reviews have reported biomaterials and biomedical devices with antibiofouling properties against other biomacromolecule adherence from physiological fluids or implanting sites.Citation8,Citation15,Citation48,Citation86–93 But reviews dealing with antibacterial, antibiofilm, and antibiofouling properties simultaneously against microbial species and biomacromolecules are not much explored yet, to the authors’ best knowledge. In this review, we comprehensively discussed biomaterials and biomedical devices with these three properties: antibacterial, antibiofilm, and antibiofouling simultaneously, not only against microbial species but also against the adhesion of other biomacromolecules, which distinguished our review from others previously published. Besides, we also recommended potential directions for further investigations.
Recent Progress and Different Strategies for the Fabrication of Antibacterial, Antibiofilm, and Antibiofouling Biomaterials and Biomedical Devices
Significant research has been conducted to develop antimicrobial and antibiofouling biomaterials and biomedical devices. To develop biomaterials and biomedical devices with superior antibacterial, antibiofilm, and antibiofouling properties, their surfaces can be functionalized with a variety of biologically active chemical substances capable of killing and inhibiting pathogenic bacteria from forming biofilms, as well as reducing protein, platelet, and live/dead microbe adsorption/adhesion.Citation8 These characteristics may be obtained either by functionalizing a single molecule or by combining two or three molecules. Each molecule performs a distinct function: antibacterial, biofilm formation inhibitor, and biomacromolecules (live/dead bacterial cells, proteins, and platelets) adhesion inhibitor. Antimicrobial activity can be accomplished primarily either by (i) releasable bactericidal (release killing) or (ii) the inclusion of non-releasable bactericidal (contact killing) near the surface. To inhibit adhesion, antibiofouling agents typically use one of three mechanisms: (i) steric repulsion/hydration (repelling), (ii) specific protein interactions, or (iii) low surface energy (releasing) ().Citation1,Citation94
Figure 7 Biomaterial’s infection prevention strategies.
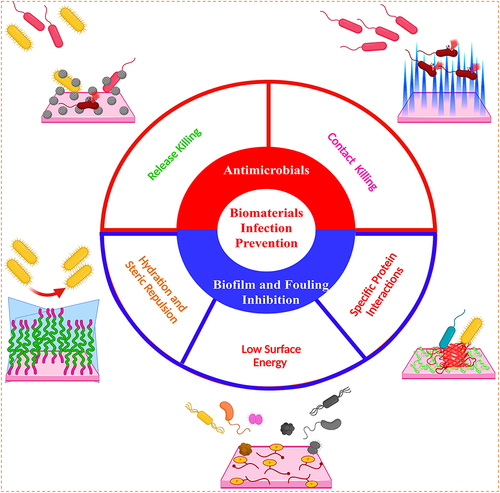
Antimicrobial Agents
Incorporating bactericidal materials/reagents onto a surface and conducting antimicrobial activities via release or contact killing is the most common antimicrobial approach,Citation2,Citation95,Citation96 and based on respective approaches, they are known as releasable and non-releasable bactericidal molecules, respectively. The availability of a wide variety of bactericidal molecules, many of which have been authorized by regulatory authorities and are ready to be tailored for specific applications, is an apparent benefit of this method.Citation2
Release Killing Approach
In a release killing approach, releasable bactericidal molecules are released from the coatings present on the surface of biomaterials and biomedical devices into the solid/liquid interface and liquid phase, resulting in a toxic atmosphere for pathogenic microbial species. Moreover, the concentration of releasable bactericidal can easily be increased from its minimal inhibitory concentration on the surface, leading to effectively killing the invading microbial species and further preventing the surface from contamination.Citation2,Citation94 However, the releasing approach has adverse effects on polluting the water and surrounding environment, resulting in the development of drug resistance in bacteria and fungi. Moreover, after the consumption of releasable bactericidal from the surface, biomaterials and biomedical devices can no longer have antimicrobial efficacy, and the chances of contamination rise.Citation2,Citation97–99 In this instance, reloading of releasable bactericidal molecules is further required for sustained and long-term antimicrobial properties.
Contact Killing Approach
The contact killing approach relies on non-releasable bactericidal molecules that are deadly to pathogenic bacteria being attached to the surface of biomaterials and biomedical devices.Citation1,Citation100,Citation101 In this approach, non-releasable bactericidal molecules are usually immobilized either by covalent or non-covalent interactions. Being surface immobilized, these molecules demonstrate non-leaching behavior, which further enables them to express continuous and long-term antimicrobial performance. Additionally, as a result of these features, this method is regarded as ecologically beneficial since it eliminates the need for reloading bactericidal molecules, as opposed to the release killing approach.Citation100,Citation102–105
Despite these distinguishing characteristics, the contact killing approach has been found to have limitations. Numerous studies have shown the deposition of dead microbiological debris and foulants on the surface of biomaterials and biomedical devices after their antimicrobial performance, further masking the contact active functionalities and resulting in decreased killing effectiveness. Additionally, adsorbed dead microbiological debris also contributes significantly to the attachment and transformation of live bacteria into microbial biofilms.Citation100
Combination of Releasable and Non-Releasable Bactericidal for Modifying the Biomaterial’s Surfaces
Recent studies demonstrate that metal nanoparticles can be immobilized on titanium implants via plasma immersion implantation to exhibit long-lasting antibacterial and antibiofilm properties against a variety of surface adherent bacteria in vitro/in vivo without the development of MDR bacteria or cytotoxicity.Citation106–108 However, methicillin-resistant S. aureus and S. aureus have been reported to develop robust biofilms consisting of free-floating aggregates in human synovial fluid and colonization in peri-implant tissues, respectively considered common phenomena for implant infections.Citation109–112 Thus, traditional contact killing interface methods may be ineffective in inhibiting free-floating biofilms or microbial colonization in peri-implant tissues and therefore may not meet therapeutic requirements.Citation106,Citation113,Citation114 Therefore, surface functionalization of biomaterials implants using a combination of contact-killing (non-releasable) and release-killing (releasable) bactericidal agents may be an alternate and feasible approach for preventing free-floating aggregates and microbial fouling, colonization, and biofilm formation.Citation113 Either of metals (silver, gold, and copper), metal oxides (ZnO, and CuO), doped materials (Cu-doped ZnO), small molecules, pH triggered the collapse of grafted antifouling chains, enzyme degradable layer-by-layer assemblies of alternating bactericidal and repelling polymers, low-fouling zwitterionic surface moieties, and quaternary ammonium compounds can be utilized as contact killing or release killing bactericidal depending on the coating methodology, coating substrate, and desired applications.
One such approach is shown in , in which Wang et al developed titanium-based biomaterials that include bactericidal moieties capable of contact and release killing. To create a contact-killing surface, Ag nanoparticles are implanted into titania (TiO2) nanotubes through anodic oxidation and plasma immersion ion implantation. On the other hand, Vancomycin is subsequently vacuum extracted and lyophilized into the nanotubes to create the release-killing effect (). The vancomycin-loaded and silver-implanted TiO2 nanotubular surface exhibits better antibacterial and antibiofilm action against planktonic/adherent bacteria without eliciting substantial silver ion release. Additionally, the synergetic combination of contact-and release-killing activities, as well as cell-assisting functions, enables the development of a novel and effective strategy for preventing bacterial infection and biofilm formation on biomaterials, with great potential for orthopaedic applications ().Citation113
Figure 8 (A) The three-step method of (i) anodic oxidation to produce the TiO2 nanotubes array, (ii) plasma immersion ion implantation to embed Ag nanoparticles into the TiO2 nanotubes, and (iii) vacuum extraction to loading vancomycin into the TiO2 nanotubes is shown schematically; (B) antibacterial activity in the bacteria/cells coculture model: SEM (scanning electron microscope) morphologies of bacteria and fibroblasts linked to the four samples. The photographs below are magnified more than the regions indicated by the red boxes. The bacteria are denoted by the blue arrow, whereas the green arrow marks the fibroblast; (C) fluorescence pictures of fibroblasts stained with DAPI (4′,6-diamidino-2-phenylindole) (blue) and TRITC (Tetramethylrhodamine isothiocyanate)-phalloidin (red) on four samples; (D) surface covers four distinct surfaces following three days of fibroblast cell attachment, spreading, and maturation (**P < 0.01, ***P < 0.001.); (E) schematic representation of the bacteria, fibroblasts, and sample processes. In vivo assessment of peri-implant soft tissues in four groups; (F) X-ray pictures of rabbits 15 days after surgery with different implants. The red oval indicates soft-tissue swelling, and groups NT-V and NT-Ag-V have little or no infectious symptoms; (G) inflammation of the peri-implant soft tissues was seen in all four groups; (H) gross scores of inflammation in the peri-implant soft tissues in four groups (***P < 0.001); (I) bacterial survival in soft tissues around the washer (*P < 0.05, **P < 0.01, ***P < 0.001); (J) a schematic depicting the antibacterial properties of the proposed surface, which has release-killing, contact-killing, and trap-killing capabilities; and (K) illustration depicting potential antibacterial and antibiofilm strategies in a concurrent cell-bacteria system on the titanium surface.
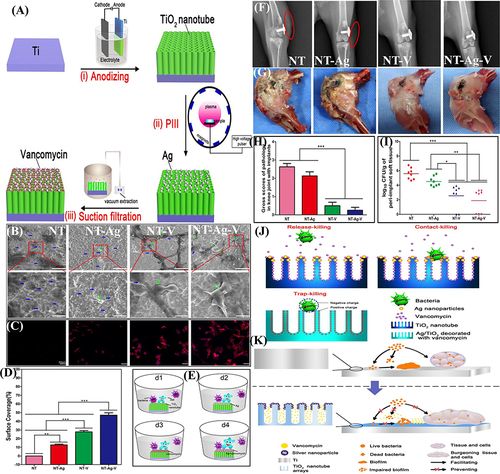
To combat Gram-positive, Gram-negative, and MDR microbial infections, the combination of contact killing and release killing bactericidal approaches appeared more promising than either strategy alone.Citation113 Despite the fact that this technique showed outstanding inhibitory efficacy against a variety of microbially contaminated implants, the possibility of biomaterial implants fouling with platelets and other macromolecules (proteins) still exists. In this case, the most recommended approach for addressing these challenges in one unit is to combine contact and release killing bactericidal approach with foul repelling/releasing antibiofouling agents.
Foul Repelling Antibiofouling Agents
Antibiofouling agents such as anionic polymers (hyaluronic acid, heparin, etc), nonionic hydrophilic polymers (polyethylene glycol, poly (2-hydroxyethyl methacrylate), poly(acrylamide), poly(vinylpyrrolidone), certain natural polysaccharides (agarose, etc) and zwitterionic polymers (sulfobetaines, carboxybetaines, phosphonobetaines, etc) generally follow the steric repulsion or hydration mechanism for repelling microbial species (live/dead) and biomacromolecules from their attachment to the surface of biomaterials and biomedical devices.Citation1,Citation87,Citation91,Citation100,Citation115–124 They develop strong hydration layers (ionically solvated) on the surfaces of biomaterials and biomedical devices with the surrounding water through hydrogen bonding. This hydration layer further develops a physical and thermodynamically barrier against the adhesion of microbial species ().Citation1,Citation100 Additionally, they can inhibit bacterial adherence through steric repulsion, which occurs when an invading bacterium compresses surface-grafted polymer chains, decreasing the number of possible chain conformations and resulting in an entropically unfavorable state ().Citation119,Citation125
Foul Releasing Antibiofouling Agents
On the other hand, antibiofouling agents with low surface energies accomplish minimal fouling by using the principles of surface free energy and adhesion work.Citation1 Their coatings on the surface of biomaterials and biomedical devices generally work by minimizing the adhesion of invading bacteria (live/dead) and biomacromolecules through hydrodynamic shear or a simple mechanical cleaning step ().Citation1,Citation100,Citation125 The majority of low surface energy antibiofouling agents are hydrophobic polymers; fluoropolymers such as poly(tetrafluoroethylene) and silicones such as poly(dimethylsiloxane) have been explored extensively because they possess among the lowest of surface energies (<25 mN/m), chemical and thermal stability, and are presumably bioinert.Citation1,Citation126,Citation127 While poly(tetrafluoroethylene) was among the first fluorinated polymers studied for fouling-release coatings, it was shown to be sensitive to shear breakdown, resulting in rapid and irreversible fouling. Moreover, poly(tetrafluoroethylene) is also challenging to process because of its low solubility and strong crystallinity.Citation100,Citation128 Other fluorinated polymers, such as fluorinated (meth)acrylates and perfluoropolyethers, have been shown to be useful in fouling-release applications, as evidenced by coatings with fluorinated comb-shaped liquid-crystalline block copolymers that showed microorganism-dependent antifouling activity.Citation129 Among silicone-based polymers, poly(dimethylsiloxane) has been widely investigated as a fouling-release coating.Citation100,Citation130,Citation131
Integrating the Bactericidal Molecules (Releasable/Non-Releasable) with Antibiofouling Agents
Given the synergistic benefits of bactericidal and antibiofouling coatings, integrating these two functions into a single coating on the surfaces of biomaterials and biomedical devices facilitates the approach to developing the promising antibacterial coating.Citation100
Combination of Bactericidal (Releasable) and Antibiofouling Agents for Modifying the Biomaterial and Biomedical Device’s Surfaces
This combinatorial strategy is generally called release killing + foul repelling and release killing + foul releasing depending on the integrated antibiofouling agent. In this combinatorial strategy, bactericidal provides the first line of defense to the surfaces of biomaterials and biomedical devices by releasing against the planktonic pathogenic Gram-positive, Gram-negative, and multidrug-resistant (MDR) bacterial strains.Citation2 These bactericidal can have a different killing mode of action that depends upon their nature and molecular functionalities. However, the mechanisms most commonly suggested are as follows: (i) transcriptional arrest, (ii) loss of membrane integrity, (iii) protein dysfunction, and (iv) induced oxidative stress.Citation132,Citation133 The second line of defense is provided by the antibiofilm agents when some pathogenic bacterial strains can be succeeded in saving their lives from the bactericidal action. The remaining alive bacteria readily tend to convert into biofilms in response to bactericidal stress. Therefore, these biofilm inhibiting agents impede the transformation of microbes into biofilms on the surfaces of biomaterials and biomedical devices. After the action of bactericidal and antibiofilm agents, some dead or alive bacteria remain on the biomaterial’s surface. These bacteria can further act as a platform for the adhesion and proliferation of the new bacteria. In this scenario, the third line of defense is provided by the antibiofouling agents by repelling or releasing these bacteria from accumulating on the surfaces of biomaterials and biomedical devices.Citation2
The releasable biocidal agents in this strategy are generally metals (silver, gold, and copper), metal oxides (ZnO and CuO), doped materials (Cu-doped ZnO), nitric oxides, antibiotics, and small molecules.Citation134–138 At the same time, antibiofouling agents can be foul repelling/releasing or a combination of both. They can be nonionic, Zwitterionic, hydrophilic, or hydrophobic, and their choice is usually made on the type of protein and finally on the application of biomaterials and biomedical devices.Citation100 The shelf life of this strategy depends on the releasing rate of bactericidal molecules. If the releasing rate of these molecules is so high, then this strategy has a short shelf life and vice versa.Citation1 In this combinatorial strategy, interferences of these bactericidal with antibiofilm and antibiofouling agents appeared very minimal due to their release.Citation2 This combinatorial strategy is considered beneficial due to its continual and streamlined antibacterial performance by killing bacteria, inhibiting biofilm formation, and prohibiting the biofouling of live/dead bacteria, proteins, and platelets.Citation2
Using this approach, Singha et al developed a remarkably effective antifouling hydrophilic biomedical grade coating for nitric oxide (NO) releasing hydrophobic polymers. The coating substantially decreased protein adhesion and biofilm development of S. aureus under physiological conditions in vitro.Citation139 Goudie et al have further reported the liquid-infused nitric oxide-releasing material that was synthesized by a solvent swelling process incorporating silicone oil and the NO donor S-nitroso-N-acetylpenicillamine into medical-grade silicone rubber (SR). The modified silicone rubber demonstrated excellent antibiofouling properties by inhibiting the protein and platelet adhesion in vitro over a period of two hours. Further, modified silicone rubber displayed a good antibacterial and antibiofilm performances against the adhesion of S. aureus and P. aeruginosa for the period of seven days in vitro.Citation140 These two studies showed remarkable antibiofouling activity against both proteins and platelets.Citation139,Citation140
Nevertheless, it is unclear how NO can inhibit protein adherence while its antiplatelet activity is widely established.Citation141–143 As a result, further research is required to determine the NO antibiofouling effectiveness against proteins both in vitro and in vivo. Additionally, it is recommended that when NO is used as an antiplatelet and antibacterial agent to modify the surface of biomaterials and biomedical devices, it includes compounds that inhibit protein attachment.
In another study, Liu et al covalently grafted the zwitterionic terpolymer (methacryloyloxyethyl phosphorylcholine-co-butyl methacrylate-co-benzophenone) onto a silicone-polycarbonate-urethane copolymer (CarboSil) after embedding with NO donor S-nitroso-N-acetylpenicillamine. In vitro, under physiological conditions (37 °C in PBS), the resultant hydrophilic biomaterials substantially decreased protein and pathogenic bacteria adherence.Citation144 Devine et al recently modified the surface of medical-grade SR by surface immobilizing heparin (Hep) (acts as an anticoagulative agent) and incorporating the releasing bactericidal agent NO (also acts as an antiplatelet and anti-inflammatory agent) into the SR polymeric matrix (Hep-NO-SR) ().Citation70 Hep-NO-SR reduced viable S. aureus adhesion by 99.46 ± 0.17% when compared to SR in vitro adhesion tests (). When Hep-NO-SR was compared to SR, platelet adhesion was reduced by 84.12 ± 6.19% in vitro, without triggering a cytotoxic response against fibroblast cells (). Despite systemic anticoagulation, all Hep-NO-SR sustained baseline platelet count and device patency for the duration of the four-hour extracorporeal circuit model (). However, 66% of SR samples were clotted within the first two hours of the experiment. The findings indicate that Hep-NO-SR enhances the endothelium’s hemocompatibility and antibacterial capabilities by simulating two vital metabolic processes.Citation70
Figure 9 (A) Schematic demonstration for Hep (heparin) immobilization on SR (silicone rubber) followed by NO (nitric oxide) donor SNAP (S-nitroso-N-acetyl penicillamine) impregnation by swelling, resulting in a heparinized NO-releasing surface; (B) S. aureus adhesion; and (C) platelet adhesion on unmodified SR, SR modified only with Hep, SR modified only with SNAP, and SR modified with Hep and SNAP; and (D) photograph display Hep–NO–SR catheter tubes after four hours in an extracorporeal circuit model showing minimal thrombogenesis.
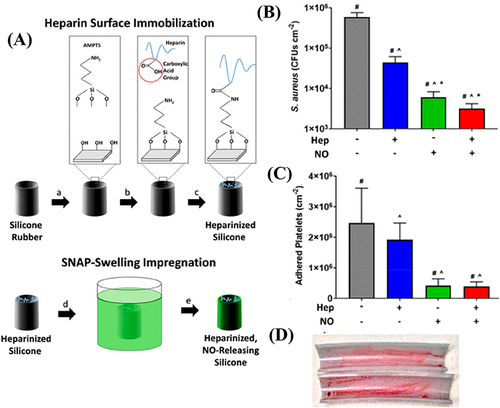
shows another example of this approach in which zwitterionic AgNPs (PCBDA@AgNPs) were developed using poly (carboxybetaine-co-dopamine methacrylamide) copolymer (PCBDA).Citation145 The PCBDA@AgNPs were immobilized on amino-modified cotton gauze (CG) through covalent and noncovalent interactions (). Due to the zwitterionic interface formed by immobilized AgNPs and the continuous release of Ag+, the as-prepared PCBDA@AgNPs-CG showed not only outstanding antiadhesive (>99.40%) and bactericidal action (>99.9% kill) against bacteria but also prevented biofilm formation even after 7 days in vitro. Additionally, this PCBDA@AgNPs-CG exhibited excellent hemocompatibility and cytocompatibility, advantageous when used as a wound dressing. More significantly, an in vivo wound healing test showed that the PCBDA@AgNPs-CG dressing could successfully inhibit wound infection, reduce chronic inflammation, and promote the healing of wounds ().
Figure 10 (A) Schematic presentation for the synthesis of PCBDA@AgNPs; (B) PCBDA@AgNPs immobilization on amino-modified cotton gauze; and (C) in vivo wound healing with the PCBDA@AgNPs-CG dressing.
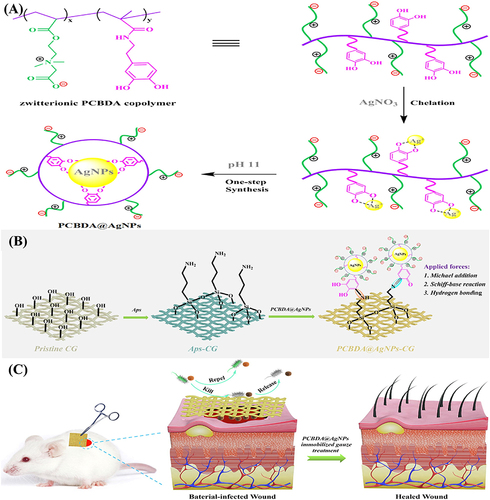
Under specific circumstances, releasable bactericidal can be reloaded; for example, Ag NPs can be periodically injected into a microbe-resistant hydrogel to maintain antibacterial activities.Citation146 These refillable surfaces may have been used for short-term application per reloading period since successful refilling needs a high diffusing capacity of the coating matrix, which leads to a more rapid release of the bactericidal from the matrix. For prolonged application, the bactericidal must be held considerably more strongly by the coating matrix, as reloading the bactericidal would be difficult, and the coating would have to be reapplied to maintain the bactericidal activities.Citation2
Combination of Bactericidal (Non-Releasable) and Antibiofouling Agents for Modifying the Biomaterial and Biomedical Device’s Surfaces
This strategy is generally referred to as contact killing + foul repelling and contact killing + foul releasing depending on the integrated antibiofouling agent. In this strategy, contact-killing active compounds include monomers, functionalized side chains, or surface-grafted functionalities that have a bactericidal effect. The bulk of bactericidal components in contact killing active biomaterials and biomedical devices are quaternary ammonium compounds, host defense peptides or analogs, natural biocidal polymers (eg, chitosan), and other cationic moieties.Citation147–151 The mode of action of these compounds is believed to be the disruption of the microbial cell wall/membrane. This is accomplished either through opposite charge attraction and subsequent penetration of the active group, resulting in the disruption of the phospholipid bilayer, or by developing an electric polarization that causes the transmembrane potential to collapse ().Citation1,Citation152–155 While antibiofouling agents can be foul repelling/releasing or a combination of both. They can be nonionic, Zwitterionic, hydrophilic, or hydrophobic, and their choice is usually made on the type of protein and finally on the application of biomaterials and biomedical devices. Examples of these include pH-triggered collapse of grafted antifouling chains, enzyme degradable layer-by-layer assemblies of alternating bactericidal and repelling polymers, low-fouling zwitterionic surface moieties, and quaternary ammonium compounds.Citation1,Citation156–161 Compared to other techniques of bactericidal release, these are non-leaching and, therefore, should not compromise their effectiveness or provide routes for developing bacterial resistance.Citation162,Citation163 Furthermore, compared to the release killing approach, the contact killing strategy has the advantage of providing long-term antimicrobial performance.Citation164–166 Numerous studies have been conducted on different synthetic techniques for the fabrication of contact-killing active biomaterials and biomedical devices based on covalent surface grafts, side chains, and cationic monomers.Citation152,Citation167
Figure 11 (A) antibacterial and antiadhesion modes of action of the contact killing strategy presented by coated non-releasable bactericidal molecules on the Titanium implant surface. Here APTES is (3-aminopropyl) triethoxysilane. Schematic presentation of antibacterial and antiadhesion performances of (B) bare dental appliance and coated with (C) polyethylene glycol (PEG) and (D) a combination of chitosan (cs) and PEG.
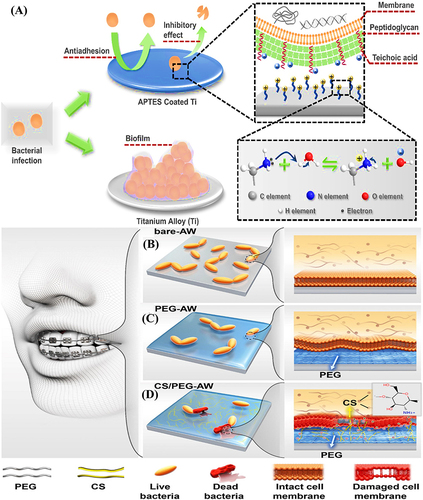
Recently, Peng et al developed an antimicrobial hydrogel that was employed for the first time in dental applications. They used a natural biocidal polymer (chitosan) as contact killing and a hydrophilic polymer (polyethylene glycol) as adhesion inhibiting. This biointerface has exceptional early-stage adhesion inhibition (98.8%, 5 h) and colony-suppression action that is remarkably long-lasting (93.3%, 7d) ().Citation168 Moreover, Zhou et al designed and synthesized the cylindrical catheter coated with non-leachable coatings comprised of three cross-linked cationic polymers (3-acrylamidopropyl) trimethylammonium chloride or quaternized polyethylenimine methacrylate together with polyethylene glycol dimethacrylate. The coated catheter demonstrated excellent antibacterial and antibiofilm performance both in vitro as well as in vivo in the mouse catheter model against the vancomycin-resistant E. faecalis and methicillin-resistant S. aureus.Citation169 Although both the coated dental appliance and the coated cylindrical catheter demonstrated noteworthy antibacterial, antibiofilm, and antiadhesion performance,Citation168,Citation169 but they were not evaluated for the adhesion of other biomacromolecules present in the implanting sites and physiological fluids that can potentially cause serious adverse effects.
Using antimicrobial peptides and polyethylene glycol, Li et al developed and synthesized diblock amphiphilic compounds having antibacterial and antifouling properties. Additionally, the synthesized copolymers were grafted onto the surfaces of silicone rubber (a commonly used catheter material), resulting in an excellent coating. The coating developed on silicone rubber from the copolymers demonstrated exceptional bactericidal activity (in vitro and in vivo) against pathogenic bacteria (S. aureus, P. aeruginosa, and E. coli) via contact killing strategy and excellent antifouling performance for decreasing bacteria, protein, and platelet adsorption ().Citation170 Additionally, polymer brushes incorporating antimicrobial peptides can be utilized to develop self-adaptive antimicrobial surfaces for catheters, as well as antifouling and antibacterial coatings.Citation171,Citation172 Xu et al, for example, developed polymer brushes that are antifouling, antibacterial, and hemocompatible. This coating showed excellent antibacterial performance via contact killing strategy against both Gram-positive and Gram-negative bacteria and further significantly prevented the bacterial debris and bovine serum albumin adsorption on the surface of PU-DMH. Moreover, in vivo experiment results demonstrate that PU-DMH can prohibit catheter-induced bacterial infections, exhibiting anti-infection performances ().Citation172
Figure 12 (A) A schematic representation of the antibacterial and antibiofouling properties of silicone rubber tubes (a commonly used catheter material) coated with copolymers; rat implanted with (B and C) pristine PDMS (left) and g-PEG45-b-AMP coated PDMS (right) at 0 and 5th day, respectively; (D and E) rat images after implants removal for antiadhesion test against bacterial adhesion at 5th day; (F) Log CFU/implant; (G) representative colonies images of E. coli isolated from both implants; and (H) SEM images of adhered bacteria on both implants. (I) schematic presentation for the molecular structure and effects of PU-DMH; (J) schematic demonstration for the antibacterial performance evaluation under flow conditions; (K) confocal laser scanning microscopic images after circulating experiment; and (L) S. aureus density on PU and PU-DMH surfaces.
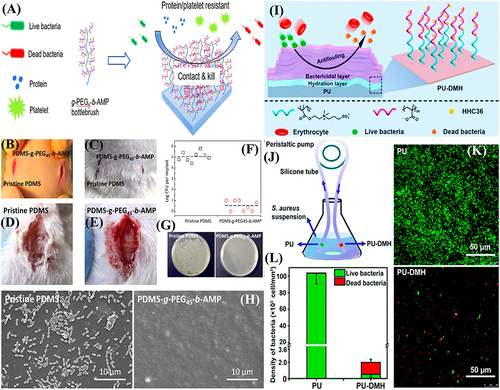
and ) is another example of this combinatorial strategy in which a zwitterionic and antimicrobial Cu metal-phenolic network was developed, and further, contact lenses were coated.Citation173 The coated contact lenses demonstrated (> 99.0%) antiadhesion and (> 99.9%) broad-spectrum antibacterial performance against all tested pathogenic bacterial species via contact killing strategy (). The coated contact lenses displayed good biofilm inhibition efficacy for 14 days in the microbial environment and exhibited excellent antibiofouling propensity against bovine serum albumin (). Furthermore, Shen et al demonstrated that coating the medical-grade titanium surface with (3-aminopropyl) triethoxysilane (), which has a hydrophobic alkyl chain and a positively charged amino group, prevented more than 90% of the initial adhesion of E. coli, P. aeruginosa, and S. aureus in vitro antimicrobial testing. After 24-hour culture, the treated surface was able to inhibit 75.7% of bacterial growth, demonstrating excellent biofilm inhibition performance. When the coated titanium was implanted into a rat model infected with S. aureus, the implant-associated infection was eradicated via the mechanism of inhibition of bacterial adhesion on the implant surface.Citation166
Figure 13 (A) Schematic illustration for the synthesis of zwitterionic antimicrobial Cu metal-phenolic network and coating of contact lens; (B) antibacterial and antifouling mechanism; (C) antiadhesion; (D) antibacterial; and (E) antibiofouling propensities. Reproduced with permission.Citation173 (F) schematic presentation for the medical-grade titanium surface coated with (3-aminopropyl) triethoxysilane. Reproduced with permission.Citation166
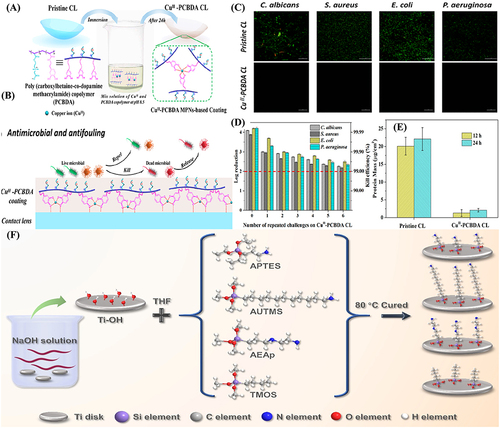
When the non-releasable bactericidal moieties combine with the fouling repelling/releasing properties having materials for surface coating, both materials tend to interact with the invasive pathogenic microbial species depending on their chemical and physical properties (hydrophobicity/hydrophilicity, size, and chain length). Both may or may not compromise the killing and fouling repelling/releasing functions of each due to differences in their spatial-temporal presence on the surface.Citation2,Citation174–176 For instance, non-releasable bactericidal moieties tend to interact with microbial species for killing. On the other hand, foul repelling materials be inclined to repel them simultaneously. Therefore, co-existence on the biomaterial surface leads to compromise their antibacterial and antifouling performances (). However, when the non-releasable bactericidal and fouling releasing materials co-existed on the biomaterial’s surface, the chances of compromising their antibacterial and antifouling performance are very minimal as both tend to interact with pathogenic microbials to some extent ().Citation2 However, contradictory reports are available combining the foul releasing antibiofouling agents with the non-releasable bactericidal. This is because of the fact that bacteria adhere to non-releasable bactericidal very strongly through electrostatic force of attraction and are difficult to release by the application of shear force.Citation100 Furthermore, mostly cationic polymers are used as non-releasable bactericidal agents that are soluble in water and hydrophilic in nature. On the other hand, fluorinated polymers or silicone-based elastomers used for foul releasing are insoluble in water due to their hydrophobicity. As a consequence, combining these components with such differential characteristics during coating manufacturing may result in phase separation.Citation100 Furthermore, when the non-releasable bactericidal and fouling repelling materials are not on the same surface level, as shown in , where the fouling repelling material is immobilized at the outer surface, and the non-releasable bactericidal is closer to the substrate, the compromise in antibacterial and antifouling efficiency may be marginal.Citation2
Figure 14 (A) Interaction of co-existing non-releasable bactericidal and fouling repelling moieties with microbials on biomaterial surface; (B) Interaction of co-existing non-releasable bactericidal and fouling releasing moieties with microbials on biomaterial surface; and (C) Interaction of co-existing non-releasable bactericidal and fouling repelling moieties with microbials on the surfaces of biomaterials and biomedical devices.
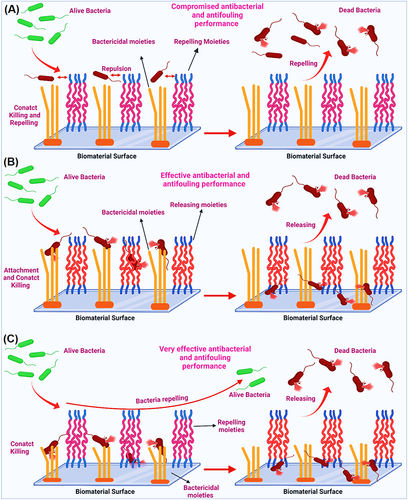
The combination approach’s unique architecture allows external stimuli to regulate the temporal existence of the two materials, permitting just one kind of material to emerge and operate at a time to resolve potential compromise. Immobilized cationic polymers, for example, have been used to produce an antimicrobial surface that exerts bactericidal effects on adhering bacteria. Following a particular treatment to hydrolyze a portion of the polymers, the surface generated zwitterionic carboxybetaine, allowing previously contact-killed bacteria to be freed and/or readily eliminated.Citation177 Furthermore, a new structured polymer surface may permit infinite swapping of zwitterionic polymers and cationic polymers via reversible ester bond formation/breaking activated by different pH conditions, executing either microbicidal or resistant functions as needed.Citation59 In order to govern the spatial-temporal existence of the two materials in a particular application that requires additional validation, it must be advised that some external adaptation is necessary.
Conclusion and Future Remarks
The high financial costs and human suffering associated with the biomaterials and biomedical device-induced infections caused by their microbial contamination and biomacromolecules adhesions motivated the development of antibacterial, antibiofilm, and antibiofouling coatings. To tackle these infestations, antibacterial, antibiofilm, and antibiofouling coatings on biomaterials and biomedical devices must not only be very effective in preventing bacterial colonization and subsequent biofilm formation but also have the ability to prohibit the adhesion of other biomacromolecules (platelets, proteins, etc). Therefore, the fabrication of dual-functional antibiofouling and bactericidal coatings on biomaterials and biomedical devices is considered highly promising in this instance to prohibit microbial infections and adverse biological effects (thrombus, fibrosis, etc). Polymeric molecules are considered the base units for the generation of such dual-functional coatings on biomaterials and biomedical devices owing to their adjustable physicochemical characteristics, and further, depending on the requirements of biomedical applications, their design can be tuned. While numerous antibiofouling and/or bactericidal coatings have been reported individually, much less research has targeted the integration of antibacterial and antibiofouling coatings in one unit on biomaterials and biomedical devices tailored for specific particular biological purposes.
Developing dual-functional coatings on biomaterials and biomedical devices for its desired application is critical, not only for its effectiveness and durability throughout the specified time of the application but also for maintaining the original device/intended surface’s functionality. For instance, excessive concentrations of cationic bactericidal polymers may increase platelet activation and hemolysis in a blood-contact environment, and antibiofouling coatings can impair the osseointegration of orthopedic implants. It is reasonable to anticipate that the optimum amounts of antibiofouling and bactericidal molecules in the coating required for good effectiveness will vary across biomedical applications.
Conversely, even a well-designed dual-functional coating on biomaterials and biomedical devices that performs well in the laboratory environmental conditions may have difficulties or can fail to demonstrate similar efficacy during clinical therapeutic application for many reasons.Citation100 For example, dual-functional coating on biomaterials and biomedical devices in the laboratory is mainly evaluated on a shorter time scale than practical systems, leaving unaddressed problems of long-term coating effectiveness and durability, as well as possible cytotoxic consequences. If releasable bactericidal drugs are to be utilized for extended periods of time, prolonged-release is essential to achieving antibacterial effectiveness while minimizing toxicity. Another factor is that most of the fabricated dual-functional coatings on biomaterials and biomedical devices are evaluated in vitro, where the human body’s complex physiological and biological milieu cannot be easily replicated. In this instance, one possible solution is integrating surface-functionalized approaches with 3D printing, which further broadens the window of antibacterial and antibiofouling coatings on biomaterials and biomedical devices.Citation100 Novel antibacterial and antibiofouling coatings will continue to be developed and applied to biomaterials and biomedical devices. However, for effective and practical applications to avoid hospital-acquired infections, academic researchers will need to consider manufacturing and clinical perspectives in their designs to increase the likelihood of their products reaching the clinic and making an impact in reducing hospital-acquired infections.
Disclosure
The authors declared no conflicts of interest.
Acknowledgment
This publication is based upon work supported by King Fahd University of Petroleum & Minerals, and the author at KFUPM acknowledges the support. We also thank PolyU Distinguished Postdoctoral Fellowship Scheme at The Hong Kong Polytechnic University, Hong Kong.
References
- Zander ZK, Becker ML. Antimicrobial and antifouling strategies for polymeric medical devices. ACS Macro Lett. 2018;7(1):16–25. doi:10.1021/ACSMACROLETT.7B00879
- Song B, Zhang E, Han X, Zhu H, Shi Y, Cao Z. Engineering and application perspectives on designing an antimicrobial surface. ACS Appl Mater Interfaces. 2020;12(19):21330–21341. doi:10.1021/ACSAMI.9B19992
- Costerton JW, Stewart PS, Greenberg EP. Bacterial biofilms: a common cause of persistent infections. Science. 1999;284(5418):1318–1322. doi:10.1126/SCIENCE.284.5418.1318
- Magill SS, Edwards JR, Bamberg W, et al. Multistate point-prevalence survey of health care–associated infections. N Engl J Med. 2014;370(13):1198–1208. doi:10.1056/NEJMOA1306801
- Haque M, Sartelli M, McKimm J, Bakar MA. Health care-associated infections – an overview. Infect Drug Resist. 2018;11:2321–2333. doi:10.2147/IDR.S177247
- Pronovost P, Needham D, Berenholtz S, et al. An intervention to decrease catheter-related bloodstream infections in the ICU. N Engl J Med. 2009;355(26):2725–2732. doi:10.1056/NEJMOA061115
- O’Grady NP, Alexander M, Dellinger EP, et al. Guidelines for the prevention of intravascular catheter–related infections. Clin Infect Dis. 2002;35(11):1281–1307. doi:10.1086/344188
- Damodaran VB, Murthy NS. Bio-inspired strategies for designing antifouling biomaterials. Biomater Res. 2016;20(1):1–11. doi:10.1186/S40824-016-0064-4
- Khan SA, Lee CS. Recent progress and strategies to develop antimicrobial contact lenses and lens cases for different types of microbial keratitis. Acta Biomater. 2020;113:101–118. doi:10.1016/j.actbio.2020.06.039
- Khan SA, Shahid S, Mahmood T, Lee C-S. Contact lenses coated with hybrid multifunctional ternary nanocoatings (Phytomolecule-coated ZnO nanoparticles:Gallic Acid:Tobramycin) for the treatment of bacterial and fungal keratitis. Acta Biomater. 2021;128:262–276.
- Marrie TJ, Nelligan J, Costerton JW. A scanning and transmission electron microscopic study of an infected endocardial pacemaker lead. Circulation. 1982;66(6 I):1339–1341. doi:10.1161/01.CIR.66.6.1339
- Lebeaux D, Ghigo J-M, Beloin C. Biofilm-related infections: bridging the gap between clinical management and fundamental aspects of recalcitrance toward antibiotics. Microbiol Mol Biol Rev. 2014;78(3):510–543. doi:10.1128/MMBR.00013-14
- Donlan RM, Costerton JW. Biofilms: survival mechanisms of clinically relevant microorganisms. Clin Microbiol Rev. 2002;15(2):167–193. doi:10.1128/CMR.15.2.167-193.2002
- Lebeaux D, Ghigo JM. Management of biofilm-associated infections: what can we expect from recent research on biofilm lifestyles? Med Sci. 2012;28(8–9):727–739. doi:10.1051/medsci/2012288015 French.
- Ahmed W, Zhai Z, Gao C. Adaptive antibacterial biomaterial surfaces and their applications. Mater Today Bio. 2019;2:100017. doi:10.1016/J.MTBIO.2019.100017
- Perni S, Preedy EC, Prokopovich P. Success and failure of colloidal approaches in adhesion of microorganisms to surfaces. Adv Colloid Interface Sci. 2014;206:265–274. doi:10.1016/J.CIS.2013.11.008
- Ribeiro M, Monteiro FJ, Ferraz MP. Infection of orthopedic implants with emphasis on bacterial adhesion process and techniques used in studying bacterial-material interactions. Biomatter. 2012;2(4):176–194. doi:10.4161/BIOM.22905
- Nguyen VT, Chia TWR, Turner MS, Fegan N, Dykes GA. Quantification of acid–base interactions based on contact angle measurement allows XDLVO predictions to attachment of Campylobacter jejuni but not Salmonella. J Microbiol Methods. 2011;86(1):89–96. doi:10.1016/J.MIMET.2011.04.005
- Arciola CR, Campoccia D, Montanaro L. Implant infections: adhesion, biofilm formation and immune evasion. Nat Rev Microbiol. 2018;16(7):397–409. doi:10.1038/s41579-018-0019-y
- Kline KA, Fälker S, Dahlberg S, Normark S, Henriques-Normark B. Bacterial adhesins in host-microbe interactions. Cell Host Microbe. 2009;5(6):580–592. doi:10.1016/J.CHOM.2009.05.011
- Mandlik A, Swierczynski A, Das A, Ton-That H. Pili in Gram-positive bacteria: assembly, involvement in colonization and biofilm development. Trends Microbiol. 2008;16(1):33–40. doi:10.1016/J.TIM.2007.10.010
- Bose JL, Lehman MK, Fey PD, Bayles KW. Contribution of the Staphylococcus aureus Atl AM and GL Murein Hydrolase Activities in Cell Division, Autolysis, and Biofilm Formation. PLoS One. 2012;7(7):e42244. doi:10.1371/JOURNAL.PONE.0042244
- Foster SJ. Molecular characterization and functional analysis of the major autolysin of Staphylococcus aureus 8325/4. J Bacteriol. 1995;177(19):5723–5725. doi:10.1128/JB.177.19.5723-5725.1995
- Heilmann C, Hussain M, Peters G, Götz F. Evidence for autolysin-mediated primary attachment of Staphylococcus epidermidis to a polystyrene surface. Mol Microbiol. 1997;24(5):1013–1024. doi:10.1046/J.1365-2958.1997.4101774.X
- Franz S, Rammelt S, Scharnweber D, Simon JC. Immune responses to implants – a review of the implications for the design of immunomodulatory biomaterials. Biomaterials. 2011;32(28):6692–6709. doi:10.1016/J.BIOMATERIALS.2011.05.078
- Speziale P, Pietrocola G, Rindi S, et al. Structural and functional role of Staphylococcus aureus surface components recognizing adhesive matrix molecules of the host. Future Microbiol. 2009;4(10):1337–1352. doi:10.2217/FMB.09.102
- Dunne WM. Bacterial adhesion: seen any good biofilms lately? Clin Microbiol Rev. 2002;15(2):155. doi:10.1128/CMR.15.2.155-166.2002
- Campoccia D, Speziale P, Ravaioli S, et al. The presence of both bone sialoprotein-binding protein gene and collagen adhesin gene as a typical virulence trait of the major epidemic cluster in isolates from orthopedic implant infections. Biomaterials. 2009;30(34):6621–6628. doi:10.1016/J.BIOMATERIALS.2009.08.032
- Costerton JW, Montanaro L, Arciola CR. Biofilm in Implant Infections: its Production and Regulation. Int J Artif Organs. 2018;28(11):1062–1068. doi:10.1177/039139880502801103
- Arciola CR, Campoccia D, Ravaioli S, Montanaro L. Polysaccharide intercellular adhesin in biofilm: structural and regulatory aspects. Front Cell Infect Microbiol. 2015;1:7. doi:10.3389/FCIMB.2015.00007
- Arciola CR, Campoccia D, Gamberini S, et al. Antibiotic resistance in exopolysaccharide-forming Staphylococcus epidermidis clinical isolates from orthopaedic implant infections. Biomaterials. 2005;26(33):6530–6535. doi:10.1016/J.BIOMATERIALS.2005.04.031
- Fedtke I, Mader D, Kohler T, et al. A Staphylococcus aureus ypfP mutant with strongly reduced lipoteichoic acid (LTA) content: LTA governs bacterial surface properties and autolysin activity. Mol Microbiol. 2007;65(4):1078–1091. doi:10.1111/J.1365-2958.2007.05854.X
- Fabretti F, Theilacker C, Baldassarri L, et al. Alanine esters of enterococcal lipoteichoic acid play a role in biofilm formation and resistance to antimicrobial peptides. Infect Immun. 2006;74(7):4164–4171. doi:10.1128/IAI.00111-06
- Sabaté Brescó M, Harris LG, Thompson K, et al. Pathogenic mechanisms and host interactions in staphylococcus epidermidis device-related infection. Front Microbiol. 2017;1:1401. doi:10.3389/FMICB.2017.01401
- Cloutier M, Mantovani D, Rosei F. Antibacterial coatings: challenges, perspectives, and opportunities. Trends Biotechnol. 2015;33(11):637–652. doi:10.1016/J.TIBTECH.2015.09.002
- Lewis K. Persister cells, dormancy and infectious disease. Nat Rev Microbiol. 2006;5(1):48–56. doi:10.1038/nrmicro1557
- Flemming H-C, Wingender J, Szewzyk U, Steinberg P, Rice SA, Kjelleberg S. Biofilms: an emergent form of bacterial life. Nat Rev Microbiol. 2016;14(9):563–575. doi:10.1038/nrmicro.2016.94
- Percival SL, Suleman L, Vuotto C, Donelli G. Healthcare-associated infections, medical devices and biofilms: risk, tolerance and control. J Med Microbiol. 2015;64(4):323–334. doi:10.1099/JMM.0.000032
- Raad I. Intravascular-catheter-related infections. Lancet. 1998;351(9106):893–898. doi:10.1016/S0140-6736(97)10006-X
- Donlan RM. Biofilm Formation: a clinically relevant microbiological process. Clin Infect Dis. 2001;33(8):1387–1392. doi:10.1086/322972
- Wilson CJ, Clegg RE, Leavesley DI, Pearcy MJ. Mediation of biomaterial–cell interactions by adsorbed proteins: a review. Tissue Eng. 2005;11(1–2):1–18. doi:10.1089/TEN.2005.11.1
- Phillips KS, Patwardhan D, Jayan G. Biofilms, medical devices, and antibiofilm technology: key messages from a recent public workshop. Am J Infect Control. 2015;43(1):2–3. doi:10.1016/J.AJIC.2014.09.019
- Tambyah PA, Halvorson KT, Maki DG. A Prospective Study of Pathogenesis of Catheter-Associated Urinary Tract Infections. Mayo Clin Proc. 1999;74(2):131–136. doi:10.4065/74.2.131
- Trautner BW, Darouiche RO. Catheter-associated infections: pathogenesis affects prevention. Arch Intern Med. 2004;164(8):842–850. doi:10.1001/ARCHINTE.164.8.842
- Oliveira WF, Silva PMS, Silva RCS, et al. Staphylococcus aureus and Staphylococcus epidermidis infections on implants. J Hosp Infect. 2018;98(2):111–117. doi:10.1016/J.JHIN.2017.11.008
- Mackie G, Gao L, Yau S, Leslie DC, Waterhouse A. Clinical potential of immobilized liquid interfaces: perspectives on biological interactions. Trends Biotechnol. 2019;37(3):268–280. doi:10.1016/J.TIBTECH.2018.08.003
- Zhou H, Wang H, Niu H, Zhao Y, Xu Z, Lin T. A waterborne coating system for preparing robust, self-healing, superamphiphobic surfaces. Adv Funct Mater. 2017;27(14):1604261. doi:10.1002/ADFM.201604261
- Erathodiyil N, Chan HM, Wu H, Ying JY. Zwitterionic polymers and hydrogels for antibiofouling applications in implantable devices. Mater Today. 2020;38:84–98. doi:10.1016/J.MATTOD.2020.03.024
- Palmer J, Flint S, Brooks J. Bacterial cell attachment, the beginning of a biofilm. J Ind Microbiol Biotechnol. 2007;34(9):577–588. doi:10.1007/S10295-007-0234-4
- Xu LC, Bauer JW, Siedlecki CA. Proteins, platelets, and blood coagulation at biomaterial interfaces. Colloids Surfaces B Biointerfaces. 2014;124:49–68. doi:10.1016/J.COLSURFB.2014.09.040
- Reviakine I, Jung F, Braune S, et al. Stirred, shaken, or stagnant: what goes on at the blood–biomaterial interface. Blood Rev. 2017;31(1):11–21. doi:10.1016/J.BLRE.2016.07.003
- Cunha BA, Halton K, Graves N. Oral Versus IV Treatment for Catheter-related Bloodstream Infections - Volume 13, Number 11—November 2007 - Emerging Infectious Diseases journal - CDC. Emerg Infect Dis. 2007;13(11):1800–1801. doi:10.3201/EID1311.070729
- Neu HC. The crisis in antibiotic resistance. Science. 1992;257(5073):1064–1073. doi:10.1126/SCIENCE.257.5073.1064
- Busscher HJ, Mei van der HC, Subbiahdoss G, et al. Biomaterial-associated infection: locating the finish line in the race for the surface. Sci Transl Med. 2012;4(153):153rv10–153rv10. doi:10.1126/SCITRANSLMED.3004528
- Lehman SM, Donlan RM. Bacteriophage-mediated control of a two-species biofilm formed by microorganisms causing catheter-associated urinary tract infections in an in vitro urinary catheter model. Antimicrob Agents Chemother. 2015;59(2):1127–1137. doi:10.1128/AAC.03786-14
- H-X W, Tan L, Tang Z-W, et al. Highly Efficient Antibacterial Surface Grafted with a Triclosan-Decorated Poly(N-Hydroxyethylacrylamide) Brush. ACS Appl Mater Interfaces. 2015;7(12):7008–7015. doi:10.1021/ACSAMI.5B01210
- Nattharika A, Sabine H, Marek W. The Effectiveness of Antibiotic Activity of Penicillin Attached to Expanded Poly(tetrafluoroethylene) (ePTFE) Surfaces: a Quantitative Assessment. Biomacromolecules. 2007;8(11):3525–3530. doi:10.1021/BM700803E
- Wang W, Lu Y, Zhu H, Cao Z. Superdurable coating fabricated from a double-sided tape with long term “zero” bacterial adhesion. Adv Mater. 2017;29(34):1606506. doi:10.1002/ADMA.201606506
- Cao Z, Mi L, Mendiola J, et al. Reversibly switching the function of a surface between attacking and defending against bacteria. Angew Chemie Int Ed. 2012;51(11):2602–2605. doi:10.1002/ANIE.201106466
- Yang H, Li G, Stansbury JW, Zhu X, Wang X, Nie J. Smart Antibacterial Surface Made by Photopolymerization. ACS Appl Mater Interfaces. 2016;8(41):28047–28054. doi:10.1021/ACSAMI.6B09343
- Liu X, Chen J, Qu C, et al. A mussel-inspired facile method to prepare multilayer-agnp-loaded contact lens for early treatment of bacterial and fungal keratitis. ACS Biomater Sci Eng. 2018;4(5):1568–1579. doi:10.1021/ACSBIOMATERIALS.7B00977
- Huang J-F, Zhong J, Chen G-P, et al. A Hydrogel-Based Hybrid Theranostic Contact Lens for Fungal Keratitis. ACS Nano. 2016;10(7):6464–6473. doi:10.1021/ACSNANO.6B00601
- Silva D, de Sousa HC, Gil MH, et al. Diclofenac sustained release from sterilised soft contact lens materials using an optimised layer-by-layer coating. Int J Pharm. 2020;585:119506. doi:10.1016/J.IJPHARM.2020.119506
- Silva D, Pinto LFV, Bozukova D, Santos LF, Serro AP, Saramago B. Chitosan/alginate based multilayers to control drug release from ophthalmic lens. Colloids Surfaces B Biointerfaces. 2016;147:81–89. doi:10.1016/J.COLSURFB.2016.07.047
- Malhotra N, Bajwa SS, Joshi M, et al. Perioperative management of post-COVID-19 surgical patients: Indian Society of Anaesthesiologists (ISA National) Advisory and Position Statement. Indian J Anaesth. 2021;65(7):499. doi:10.4103/IJA.IJA_662_21
- Jaffer IH, Fredenburgh JC, Hirsh J, Weitz JI. Medical device-induced thrombosis: what causes it and how can we prevent it? J Thromb Haemost. 2015;13(S1):S72–S81. doi:10.1111/JTH.12961
- Bergqvist D, Agnelli G, Cohen AT, et al. Duration of prophylaxis against venous thromboembolism with enoxaparin after surgery for cancer. N Engl J Med. 2009;346(13):975–980. doi:10.1056/NEJMOA012385
- Quinlan DJ, McQuillan A, Eikelboom JW. Low-molecular-weight heparin compared with intravenous unfractionated heparin for treatment of pulmonary embolism: a meta-analysis of randomized, controlled trials. Ann Intern Med. 2004;140(3). doi:10.7326/0003-4819-140-3-200402030-00008
- Prat NJ, Meyer AD, Langer T, et al. Low-dose heparin anticoagulation during extracorporeal life support for acute respiratory distress syndrome in conscious sheep. Shock. 2015;44(6):560–568. doi:10.1097/SHK.0000000000000459
- Devine R, Goudie MJ, Singha P, et al. Mimicking the Endothelium: dual Action Heparinized Nitric Oxide Releasing Surface. ACS Appl Mater Interfaces. 2020;12(18):20158–20171. doi:10.1021/ACSAMI.9B22277
- Suen K, Westh RN, Churilov L, Hardidge AJ. Low-Molecular-Weight Heparin and the Relative Risk of Surgical Site Bleeding Complications: results of a Systematic Review and Meta-Analysis of Randomized Controlled Trials of Venous Thromboprophylaxis in Patients After Total Joint Arthroplasty. J Arthroplasty. 2017;32(9):2911–2919.e6. doi:10.1016/J.ARTH.2017.04.010
- Cronin RE, Reilly RF. Unfractionated Heparin for Hemodialysis: still the Best Option. Semin Dial. 2010;23(5):510–515. doi:10.1111/J.1525-139X.2010.00770.X
- Han X, Li C, Zhang S, et al. Why thromboembolism occurs in some patients with thrombocytopenia and treatment strategies. Thromb Res. 2020;196:500–509. doi:10.1016/J.THROMRES.2020.10.005
- Roberts MK, Chaney S. Heparin-induced Thrombocytopenia. J Nurse Pract. 2018;14(5):402–408.e3. doi:10.1016/J.NURPRA.2018.02.007
- Shepherd G, Mohorn P, Yacoub K, May DW. Adverse drug reaction deaths reported in United States vital statistics, 1999-2006. Ann Pharmacother. 2012;46(2):169–175. doi:10.1345/APH.1P592
- Dhakal B, Kreuziger LB, Rein L, et al. Disease burden, complication rates, and health-care costs of heparin-induced thrombocytopenia in the USA: a population-based study. Lancet Haematol. 2018;5(5):e220–e231. doi:10.1016/S2352-3026(18)30046-2
- Shanks RMQ, Donegan NP, Graber ML, et al. Heparin stimulates Staphylococcus aureus biofilm formation. Infect Immun. 2005;73(8):4596–4606. doi:10.1128/IAI.73.8.4596-4606.2005
- Biran R, Pond D. Heparin coatings for improving blood compatibility of medical devices. Adv Drug Deliv Rev. 2017;112:12–23. doi:10.1016/J.ADDR.2016.12.002
- Kocsis JF, Llanos G, Holmer E. Heparin-Coated Stents. J Long Term Eff Med Implants. 2000;10(1):19–45. doi:10.1615/JLONGTERMEFFMEDIMPLANTS.V10.I12.50
- Fukutomi M, Kobayashi S, Niwaya K, Hamada Y, Kitamura S. Changes in Platelet, Granulocyte, and Complement Activation During Cardiopulmonary Bypass Using Heparin-coated Equipment. Artif Organs. 1996;20(7):767–776. doi:10.1111/J.1525-1594.1996.TB04538.X
- Jain G, Allon M, Saddekni S, Barker J-F, Maya ID. Does Heparin Coating Improve Patency or Reduce Infection of Tunneled Dialysis Catheters? Clin J Am Soc Nephrol. 2009;4(11):1787–1790. doi:10.2215/CJN.03920609
- Tenke P, Riedl CR, Jones GL, Williams GJ, Stickler D, Nagy E. Bacterial biofilm formation on urologic devices and heparin coating as preventive strategy. Int J Antimicrob Agents. 2004;23(SUPPL. 1):67–74. doi:10.1016/J.IJANTIMICAG.2003.12.007
- Hoenicka M, Rupp P, Müller-Eising K, et al. Anticoagulation management during multivessel coronary artery bypass grafting: a randomized trial comparing individualized heparin management and conventional hemostasis management. J Thromb Haemost. 2015;13(7):1196–1206. doi:10.1111/JTH.12999
- Lobato RL, Despotis GJ, Levy JH, Shore-Lesserson LJ, Carlson MO, Bennett-Guerrero E. Anticoagulation management during cardiopulmonary bypass: a survey of 54 North American institutions. J Thorac Cardiovasc Surg. 2010;139(6):1665–1666. doi:10.1016/J.JTCVS.2010.02.038
- Warkentin T, Greinacher A. Heparin-Induced Thrombocytopenia. N Eng J Med. 2012;373:252. doi:10.3109/9781841848617
- Huang D, Wang J, Ren K, Ji J. Functionalized biomaterials to combat biofilms. Biomater Sci. 2020;8(15):4052–4066. doi:10.1039/D0BM00526F
- Faustino CMC, Lemos SMC, Monge N, Ribeiro IAC. A scope at antifouling strategies to prevent catheter-associated infections. Adv Colloid Interface Sci. 2020;284:102230. doi:10.1016/J.CIS.2020.102230
- Singha P, Locklin J, Handa H. A review of the recent advances in antimicrobial coatings for urinary catheters. Acta Biomater. 2017;50:20–40. doi:10.1016/J.ACTBIO.2016.11.070
- Mas-Moruno C, Su B, Dalby MJ. Multifunctional coatings and nanotopographies: toward cell instructive and antibacterial implants. Adv Healthc Mater. 2019;8(1):1801103. doi:10.1002/ADHM.201801103
- Neoh KG, Li M, Kang E-T, Chiong E, Tambyah PA. Surface modification strategies for combating catheter-related complications: recent advances and challenges. J Mater Chem B. 2017;5(11):2045–2067. doi:10.1039/C6TB03280J
- Mi L, Jiang S. Integrated Antimicrobial and Nonfouling Zwitterionic Polymers. Angew Chemie Int Ed. 2014;53(7):1746–1754. doi:10.1002/ANIE.201304060
- Lin P-H, B-R L. Antifouling strategies in advanced electrochemical sensors and biosensors. Analyst. 2020;145(4):1110–1120. doi:10.1039/C9AN02017A
- Lee SW, Phillips KS, Gu H, Kazemzadeh-Narbat M, Ren D. How microbes read the map: effects of implant topography on bacterial adhesion and biofilm formation. Biomaterials. 2021;268:120595. doi:10.1016/J.BIOMATERIALS.2020.120595
- Siedenbiedel F, Tiller JC. Antimicrobial polymers in solution and on surfaces: overview and functional principles. Polym. 2012;4(1):46–71. doi:10.3390/POLYM4010046
- Hu R, Li G, Jiang Y, et al. Silver–zwitterion organic–inorganic nanocomposite with antimicrobial and antiadhesive capabilities. Langmuir. 2013;29(11):3773–3779. doi:10.1021/LA304708B
- Shtykova L, Fant C, Handa P, et al. Adsorption of antifouling booster biocides on metal oxide nanoparticles: effect of different metal oxides and solvents. Prog Org Coatings. 2009;64(1):20–26. doi:10.1016/J.PORGCOAT.2008.07.005
- Adlhart C, Verran J, Azevedo NF, et al. Surface modifications for antimicrobial effects in the healthcare setting: a critical overview. J Hosp Infect. 2018;99(3):239–249. doi:10.1016/J.JHIN.2018.01.018
- Hoch M. Organotin compounds in the environment — an overview. Appl Geochemistry. 2001;16(7–8):719–743. doi:10.1016/S0883-2927(00)00067-6
- Turner A. Marine pollution from antifouling paint particles. Mar Pollut Bull. 2010;60(2):159–171. doi:10.1016/J.MARPOLBUL.2009.12.004
- Mitra D, Kang E-T, Neoh KG. Polymer-based coatings with integrated antifouling and bactericidal properties for targeted biomedical applications. ACS Appl Polym Mater. 2021;3(5):2233–2263. doi:10.1021/ACSAPM.1C00125
- Kugel A, Stafslien S, Chisholm BJ. Antimicrobial coatings produced by “tethering” biocides to the coating matrix: a comprehensive review. Prog Org Coatings. 2011;72(3):222–252. doi:10.1016/J.PORGCOAT.2011.07.004
- Mitra D, Li M, Wang R, Tang Z, Kang E-T, Neoh KG. Scalable Aqueous-Based Process for Coating Polymer and Metal Substrates with Stable Quaternized Chitosan Antibacterial Coatings. Ind Eng Chem Res. 2016;55(36):9603–9613. doi:10.1021/ACS.IECR.6B02201
- Hoque J, Akkapeddi P, Ghosh C, Uppu DSSM, Haldar J. A Biodegradable Polycationic Paint that Kills Bacteria in Vitro and in Vivo. ACS Appl Mater Interfaces. 2016;8(43):29298–29309. doi:10.1021/ACSAMI.6B09804
- Konai MM, Bhattacharjee B, Ghosh S, Haldar J. Recent progress in polymer research to tackle infections and antimicrobial resistance. Biomacromolecules. 2018;19(6):1888–1917. doi:10.1021/ACS.BIOMAC.8B00458
- Dhingra S, Joshi A, Singh N, Saha S. Infection resistant polymer brush coating on the surface of biodegradable polyester. Mater Sci Eng C. 2021;118:111465. doi:10.1016/J.MSEC.2020.111465
- Qin H, Cao H, Zhao Y, et al. In vitro and in vivo anti-biofilm effects of silver nanoparticles immobilized on titanium. Biomaterials. 2014;35(33):9114–9125. doi:10.1016/J.BIOMATERIALS.2014.07.040
- Jin G, Qin H, Cao H, et al. Zn/Ag micro-galvanic couples formed on titanium and osseointegration effects in the presence of S. aureus. Biomaterials. 2015;65:22–31. doi:10.1016/J.BIOMATERIALS.2015.06.040
- Zhao Y, Cao H, Qin H, et al. Balancing the osteogenic and antibacterial properties of titanium by codoping of mg and ag: an in vitro and in vivo study. ACS Appl Mater Interfaces. 2015;7(32):17826–17836. doi:10.1021/ACSAMI.5B04168
- Dastgheyb S, Parvizi J, Shapiro IM, Hickok NJ, Otto M. Effect of biofilms on recalcitrance of staphylococcal joint infection to antibiotic treatment. J Infect Dis. 2015;211(4):641–650. doi:10.1093/INFDIS/JIU514
- Arciola CR, Visai L, Testoni F, et al. Concise survey of staphylococcus aureus virulence factors that promote adhesion and damage to peri-implant tissues. Int J Artif Organs. 2011;34(9):771–780. doi:10.5301/IJAO.5000046
- ter Boo GJA, Grijpma DW, Moriarty TF, Richards RG, Eglin D. Antimicrobial delivery systems for local infection prophylaxis in orthopedic- and trauma surgery. Biomaterials. 2015;52(1):113–125. doi:10.1016/J.BIOMATERIALS.2015.02.020
- Broekhuizen CAN, Boer L, Schipper K, et al. Staphylococcus epidermidis is cleared from biomaterial implants but persists in peri-implant tissue in mice despite rifampicin/vancomycin treatment. J Biomed Mater Res Part A. 2008;85A(2):498–505. doi:10.1002/JBM.A.31528
- Wang J, Li J, Qian S, et al. Antibacterial Surface Design of Titanium-Based Biomaterials for Enhanced Bacteria-Killing and Cell-Assisting Functions Against Periprosthetic Joint Infection. ACS Appl Mater Interfaces. 2016;8(17):11162–11178. doi:10.1021/ACSAMI.6B02803
- Hickok NJ, Shapiro IM. Immobilized antibiotics to prevent orthopaedic implant infections. Adv Drug Deliv Rev. 2012;64(12):1165–1176. doi:10.1016/J.ADDR.2012.03.015
- Cauda F, Cauda V, Fiori C, Onida B, Garrone E. Heparin coating on ureteral double j stents prevents encrustations: an in vivo case study. J Endourol. 2008;22(3):465–472. doi:10.1089/END.2007.0218
- Kara F, Aksoy EA, Yuksekdag Z, Aksoy S, Hasirci N. Enhancement of antibacterial properties of polyurethanes by chitosan and heparin immobilization. Appl Surf Sci. 2015;357:1692–1702. doi:10.1016/J.APSUSC.2015.09.227
- Izquierdo-Barba I, Sánchez-Salcedo S, Colilla M, et al. Inhibition of bacterial adhesion on biocompatible zwitterionic SBA-15 mesoporous materials. Acta Biomater. 2011;7(7):2977–2985. doi:10.1016/J.ACTBIO.2011.03.005
- Cheng G, Zhang Z, Chen S, Bryers JD, Jiang S. Inhibition of bacterial adhesion and biofilm formation on zwitterionic surfaces. Biomaterials. 2007;28(29):4192–4199. doi:10.1016/J.BIOMATERIALS.2007.05.041
- Chen W-L, Cordero R, Tran H, Ober CK. 50th anniversary perspective: polymer brushes: novel surfaces for future materials. Macromolecules. 2017;50(11):4089–4113. doi:10.1021/ACS.MACROMOL.7B00450
- Li M, Neoh K-G, Kang E-T, Lau T, Chiong E. Surface modification of silicone with covalently immobilized and crosslinked agarose for potential application in the inhibition of infection and omental wrapping. Adv Funct Mater. 2014;24(11):1631–1643. doi:10.1002/ADFM.201302242
- Mohan T, Čas A, Bračič M, et al. Highly protein repellent and antiadhesive polysaccharide biomaterial coating for urinary catheter applications. ACS Biomater Sci Eng. 2019;5(11):5825–5832. doi:10.1021/ACSBIOMATERIALS.9B01288
- Xu G, Liu P, Pranantyo D, Neoh K-G, Kang E-T. Dextran- and Chitosan-Based Antifouling, Antimicrobial Adhesion, and Self-Polishing Multilayer Coatings from pH-Responsive Linkages-Enabled Layer-by-Layer Assembly. ACS Sustain Chem Eng. 2018;6(3):3916–3926. doi:10.1021/ACSSUSCHEMENG.7B04286
- Luo Y, Kirker KR, Prestwich GD. Cross-linked hyaluronic acid hydrogel films: new biomaterials for drug delivery. J Control Release. 2000;69(1):169–184. doi:10.1016/S0168-3659(00)00300-X
- Aicale R, Oliva F, Maffulli N, Aicale R, Oliva F, Maff N. Defensive antibacterial coating (Dac®) for prevention of infection in acl reconstruction: a feasibility study. Muscles Ligaments Tendons J. 2020;10(1):151–153. doi:10.32098/MLTJ.01.2020.22
- Maan AMC, Hofman AH, Vos de WM, Kamperman M. Recent developments and practical feasibility of polymer-based antifouling coatings. Adv Funct Mater. 2020;30(32):2000936. doi:10.1002/ADFM.202000936
- Owen MJ. Fluorosilicones. ACS Symp Ser. 2010;1051:99–108. doi:10.1021/BK-2010-1051.CH009
- Liu F, Grainger DW. 1.28 Fluorinated Biomaterials. Compr Biomater II. 2017;648–663. doi:10.1016/B978-0-12-803581-8.10148-1
- Lejars M, Margaillan A, Bressy C. Fouling Release Coatings: a Nontoxic Alternative to Biocidal Antifouling Coatings. Chem Rev. 2012;112(8):4347–4390. doi:10.1021/CR200350V
- Sitaraman K, Nick W, Christopher KO, et al. Comparison of the Fouling Release Properties of Hydrophobic Fluorinated and Hydrophilic PEGylated Block Copolymer Surfaces: attachment Strength of the Diatom Navicula and the Green Alga Ulva. Biomacromolecules. 2006;7(5):1449–1462. doi:10.1021/BM0509826
- Sadowska JM, Genoud KJ, Kelly DJ, O’Brien FJ. Bone biomaterials for overcoming antimicrobial resistance: advances in non-antibiotic antimicrobial approaches for regeneration of infected osseous tissue. Mater Today. 2021;46:136–154. doi:10.1016/J.MATTOD.2020.12.018
- Costa F, Carvalho IF, Montelaro RC, Gomes P, Martins MCL. Covalent immobilization of antimicrobial peptides (AMPs) onto biomaterial surfaces. Acta Biomater. 2011;7(4):1431–1440. doi:10.1016/J.ACTBIO.2010.11.005
- Rojas-Andrade M, Chata G. Antibacterial mechanisms of graphene-based composite nanomaterials. Nanoscale. 2017;9(3):994–1006. doi:10.1039/C6NR08733G
- Kumar S, Roy DN, Dey V. A comprehensive review on techniques to create the anti-microbial surface of biomaterials to intervene in biofouling. Colloid Interface Sci Commun. 2021;43:100464. doi:10.1016/J.COLCOM.2021.100464
- Mitra D, Kang E-T, Neoh KG. Antimicrobial copper-based materials and coatings: potential multifaceted biomedical applications. ACS Appl Mater Interfaces. 2019;12(19):21159–21182. doi:10.1021/ACSAMI.9B17815
- Rizzello L, Pompa PP. Nanosilver-based antibacterial drugs and devices: mechanisms, methodological drawbacks, and guidelines. Chem Soc Rev. 2014;43(5):1501–1518. doi:10.1039/C3CS60218D
- Jamaati H, Mortaz E, Pajouhi Z, et al. Nitric oxide in the pathogenesis and treatment of tuberculosis. Front Microbiol. 2017;1:2008. doi:10.3389/FMICB.2017.02008
- Francolini I, Vuotto C, Piozzi A, Donelli G. Antifouling and antimicrobial biomaterials: an overview. APMIS. 2017;125(4):392–417. doi:10.1111/APM.12675
- Makvandi P, Wang C, Zare EN, Borzacchiello A, Niu L, Tay FR. Metal-Based Nanomaterials in Biomedical Applications: antimicrobial Activity and Cytotoxicity Aspects. Adv Funct Mater. 2020;30(22):1910021. doi:10.1002/ADFM.201910021
- Singha P, Jitendra P. Enhanced antibacterial efficacy of nitric oxide releasing thermoplastic polyurethanes with antifouling hydrophilic topcoats. Biomater Sci. 2017;5(7):1246–1255. doi:10.1039/C6BM00948D
- Goudie MJ, Pant J, Handa H. Liquid-infused nitric oxide-releasing (LINORel) silicone for decreased fouling, thrombosis, and infection of medical devices. Sci Reports. 2017;7(1):1–13. doi:10.1038/s41598-017-14012-9
- Zang Y, Popat KC, Reynolds MM. Nitric oxide-mediated fibrinogen deposition prevents platelet adhesion and activation. Biointerphases. 2018;13(6):06E403. doi:10.1116/1.5042752
- Brisbois EJ, Major TC, Goudie MJ, Bartlett RH, Meyerhoff ME, Handa H. Improved hemocompatibility of silicone rubber extracorporeal tubing via solvent swelling-impregnation of S-nitroso-N-acetylpenicillamine (SNAP) and evaluation in rabbit thrombogenicity model. Acta Biomater. 2016;37:111–119. doi:10.1016/J.ACTBIO.2016.04.025
- Hopkins SP, Pant J, Goudie MJ, Schmiedt C, Handa H. Achieving Long-Term Biocompatible Silicone via Covalently Immobilized S-Nitroso-N-acetylpenicillamine (SNAP) That Exhibits 4 Months of Sustained Nitric Oxide Release. ACS Appl Mater Interfaces. 2018;10(32):27316–27325. doi:10.1021/ACSAMI.8B08647
- Liu Q, Singha P, Handa H, Locklin J. Covalent grafting of antifouling phosphorylcholine-based copolymers with antimicrobial nitric oxide releasing polymers to enhance infection-resistant properties of medical device coatings. Langmuir. 2017;33(45):13105–13113. doi:10.1021/ACS.LANGMUIR.7B02970
- Xiang J, Zhu R, Lang S, Yan H, Liu G, Peng B. Mussel-inspired immobilization of zwitterionic silver nanoparticles toward antibacterial cotton gauze for promoting wound healing. Chem Eng J. 2021;409:128291. doi:10.1016/j.cej.2020.128291
- Zhang D, Fu Y, Huang L, et al. Integration of antifouling and antibacterial properties in salt-responsive hydrogels with surface regeneration capacity. J Mater Chem B. 2018;6(6):950–960. doi:10.1039/C7TB03018E
- Zou Y, Zhang Y, Yu Q, Chen H. Dual-function antibacterial surfaces to resist and kill bacteria: painting a picture with two brushes simultaneously. J Mater Sci Technol. 2021;70:24–38. doi:10.1016/J.JMST.2020.07.028
- Ren H, Du Y, Su Y, Guo Y, Zhu Z, Dong A. A Review on Recent Achievements and Current Challenges in Antibacterial Electrospun N-halamines. Colloid Interface Sci Commun. 2018;24:24–34. doi:10.1016/J.COLCOM.2018.03.004
- Jiao Y, na NL, Ma S, Li J, Tay FR, Chen J. Quaternary ammonium-based biomedical materials: state-of-The-art, toxicological aspects and antimicrobial resistance. Prog Polym Sci. 2017;71:53–90. doi:10.1016/J.PROGPOLYMSCI.2017.03.001
- Kalantari K, Afifi AM, Jahangirian H, Webster TJ. Biomedical applications of chitosan electrospun nanofibers as a green polymer – review. Carbohydr Polym. 2019;207:588–600. doi:10.1016/J.CARBPOL.2018.12.011
- Alves D, Pereira MO. Mini-review: antimicrobial peptides and enzymes as promising candidates to functionalize biomaterial surfaces. Biofouling. 2014;30(4):483–499. doi:10.1080/08927014.2014.889120
- El-Refaie K, Worley SD, Broughton§ R. The chemistry and applications of antimicrobial polymers: a state-of-the-art review. Biomacromolecules. 2007;8(5):1359–1384. doi:10.1021/BM061150Q
- Ding X, Duan S, Ding X, Liu R, Xu F-J. Versatile Antibacterial Materials: an Emerging Arsenal for Combatting Bacterial Pathogens. Adv Funct Mater. 2018;28(40):1802140. doi:10.1002/ADFM.201802140
- Atefyekta S, Pihl M, Lindsay C, Heilshorn SC, Andersson M. Antibiofilm elastin-like polypeptide coatings: functionality, stability, and selectivity. Acta Biomater. 2019;83:245–256. doi:10.1016/J.ACTBIO.2018.10.039
- van de Lagemaat M, Grotenhuis A, van de Belt-Gritter B, et al. Comparison of methods to evaluate bacterial contact-killing materials. Acta Biomater. 2017;59:139–147. doi:10.1016/J.ACTBIO.2017.06.042
- Ye Z, Li S, Zhao S, Deng L, Zhang J, Dong A. Textile coatings configured by double-nanoparticles to optimally couple superhydrophobic and antibacterial properties. Chem Eng J. 2021;420:127680. doi:10.1016/J.CEJ.2020.127680
- Bieser AM, Thomann Y, Tiller JC. Contact-Active Antimicrobial and Potentially Self-Polishing Coatings Based on Cellulose. Macromol Biosci. 2011;11(1):111–121. doi:10.1002/MABI.201000306
- Jiao Y, Tay FR, Niu L, Chen J. Advancing antimicrobial strategies for managing oral biofilm infections. Int J Oral Sci. 2019;11(3):1–11. doi:10.1038/s41368-019-0062-1
- Zhou LY, Zhu YH, Wang XY, et al. Novel zwitterionic vectors: multi-functional delivery systems for therapeutic genes and drugs. Comput Struct Biotechnol J. 2020;18:1980–1999. doi:10.1016/J.CSBJ.2020.07.015
- Yan S, Shi H, Song L, et al. Nonleaching Bacteria-Responsive Antibacterial Surface Based on a Unique Hierarchical Architecture. ACS Appl Mater Interfaces. 2016;8(37):24471–24481. doi:10.1021/ACSAMI.6B08436
- Vaterrodt A, Thallinger B, Daumann K, Koch D, Guebitz GM, Ulbricht M. Antifouling and antibacterial multifunctional polyzwitterion/enzyme coating on silicone catheter material prepared by electrostatic layer-by-layer assembly. Langmuir. 2016;32(5):1347–1359. doi:10.1021/ACS.LANGMUIR.5B04303
- Walsh C. Molecular mechanisms that confer antibacterial drug resistance. Nat. 2000;406(6797):775–781. doi:10.1038/35021219
- Kohanski MA, Dwyer DJ, Collins JJ. How antibiotics kill bacteria: from targets to networks. Nat Rev Microbiol. 2010;8(6):423–435. doi:10.1038/nrmicro2333
- Zhou W, Peng X, Ma Y, et al. Two-staged time-dependent materials for the prevention of implant-related infections. Acta Biomater. 2020;101:128–140. doi:10.1016/J.ACTBIO.2019.10.023
- Chen S, Yuan L, Li Q, et al. Durable antibacterial and nonfouling cotton textiles with enhanced comfort via zwitterionic sulfopropylbetaine coating. Small. 2016;12(26):3516–3521. doi:10.1002/SMLL.201600587
- Shen J, Gao P, Han S, et al. A tailored positively-charged hydrophobic surface reduces the risk of implant associated infections. Acta Biomater. 2020;114:421–430. doi:10.1016/J.ACTBIO.2020.07.040
- Francolini I, Donelli G, Crisante F, Taresco V, Piozzi A. Antimicrobial polymers for anti-biofilm medical devices: state-of-art and perspectives. Adv Exp Med Biol. 2015;831:93–117. doi:10.1007/978-3-319-09782-4_7
- Peng L, Chang L, Si M, et al. Hydrogel-coated dental device with adhesion-inhibiting and colony-suppressing properties. ACS Appl Mater Interfaces. 2020;12(8):9718–9725. doi:10.1021/ACSAMI.9B19873
- Zhou C, Wu Y, Thappeta KRV, et al. In vivo anti-biofilm and anti-bacterial non-leachable coating thermally polymerized on cylindrical catheter. ACS Appl Mater Interfaces. 2017;9(41):36269–36280. doi:10.1021/ACSAMI.7B07053
- Gao Q, Yu M, Su Y, et al. Rationally designed dual functional block copolymers for bottlebrush-like coatings: in vitro and in vivo antimicrobial, antibiofilm, and antifouling properties. Acta Biomater. 2017;51:112–124. doi:10.1016/J.ACTBIO.2017.01.061
- Zhang Y, Zhang X, Zhao Y-Q, et al. Self-adaptive antibacterial surfaces with bacterium-triggered antifouling-bactericidal switching properties. Biomater Sci. 2020;8(3):997–1006. doi:10.1039/C9BM01666J
- Zhang X-Y, Zhao Y-Q, Zhang Y, et al. Antimicrobial peptide-conjugated hierarchical antifouling polymer brushes for functionalized catheter surfaces. Biomacromolecules. 2019;20(11):4171–4179. doi:10.1021/ACS.BIOMAC.9B01060
- Liu G, Li K, Wang H, Ma L, Yu L, Nie Y. Stable Fabrication of Zwitterionic Coating Based on Copper-Phenolic Networks on Contact Lens with Improved Surface Wettability and Broad-Spectrum Antimicrobial Activity. N Eng J Med. 2020;12(14):16125–16136. doi:10.1021/acsami.0c02143
- Wang B, Ye Z, Tang Y, et al. Fabrication of nonfouling, bactericidal, and bacteria corpse release multifunctional surface through surface-initiated RAFT polymerization. Int J Nanomedicine. 2016;12:111–125. doi:10.2147/IJN.S107472
- Ma Y, Li J, Si Y, Huang K, Nitin N, Sun G. Rechargeable antibacterial n-halamine films with antifouling function for food packaging applications. ACS Appl Mater Interfaces. 2019;11(19):17814–17822. doi:10.1021/ACSAMI.9B03464
- Caro A, Humblot V, Méthivier C, Minier M, Salmain M, Pradier C-M. Grafting of Lysozyme and/or Poly(ethylene glycol) to Prevent Biofilm Growth on Stainless Steel Surfaces. J Phys Chem B. 2009;113(7):2101–2109. doi:10.1021/JP805284S
- Cheng G, Xue H, Zhang Z, Chen S, Jiang S. A switchable biocompatible polymer surface with self-sterilizing and nonfouling capabilities. Angew Chemie Int Ed. 2008;47(46):8831–8834. doi:10.1002/ANIE.200803570