Abstract
Introduction
Although recombinant tissue plasminogen activator (rtPA) is a widely used therapy in patients with acute ischemic stroke, rtPA-induced toxicity or its adverse effects have been reported in our previous studies. However, Ginkgo biloba extract (GBE) may provide neuroprotective effects against rtPA-induced toxicity. Thus, in the present study, we investigated whether a single administration of rtPA caused neurotoxicity in the prefrontal cortex (PFC) of rats and determined whether GBE or its diterpene ginkgolide (DG) constituents were neuroprotective against any rtPA-induced toxicity.
Materials and methods
We randomly divided adult Sprague-Dawley rats into four groups that were intravenously administered saline, rtPA, rtPA+DG, or rtPA+GBE. The rats were sacrificed 24 hours later and the whole brain removed. A gas chromatography–mass spectrometry metabolomic approach was used to detect molecular changes in the PFC among the groups. Multivariate statistical and pathway analyses were used to determine the relevant metabolites as well as their functions and pathways.
Results
We found 32 metabolites differentially altered in the four groups that were primarily involved in neurotransmitter, amino acid, energy, lipid, and nucleotide metabolism. Our results indicated that a single rtPA administration caused metabolic disturbances in the PFC. Both GBE and DG effectively ameliorated these rtPA-induced disturbances, although DG better controlled the rtPA-induced glutamate and aspartate excitotoxicity and the activation of NMDA receptor.
Conclusion
Our results provide important novel mechanistic insights into the adverse effects of rtPA and offer directions for future exploration on the thrombolytic effects of rtPA combined with the administration of DG or GBE for the treatment of acute ischemic stroke in humans.
Introduction
Recombinant tissue plasminogen activator (rtPA), a serine protease, is the most effective thrombolytic treatment for patients with acute cerebral infarction.Citation1 Intravenous thrombolysis with rtPA between 3 and 4.5 hours after symptom onset can significantly improve outcomes for ischemic stroke patients.Citation2 However, in addition to its thrombolytic effect, rtPA has been associated with neuropathological effects. For instance, our previous studies indicated that rtPA can potentiate excitotoxic lesions and lead to neuronal death induced by NMDA,Citation3 as well as induce long-term anxiety-like behaviors via the ERK1/2-GAD1-GABA cascade.Citation4 Thus, more attention should be paid to the adverse neurological effects of rtPA to achieve more effective treatment of ischemic stroke.
Ginkgo biloba extract (GBE; EGb761) is a patented extract derived from the leaves of the Ginkgo biloba tree. Previous studies have investigated the neuroprotective effects of the extract on an extensive range of disorders and diseases.Citation5–Citation7 GBE and its constituent ginkgolides are potent inhibitors of platelet-activating factor, which leads to a reduction in excitotoxic damage,Citation8,Citation9 and both function as antioxidants. It has been suggested that GBE has a multitude of beneficial effects on central nervous system (CNS) function, and it has thus been widely used for treating neurologic, psychiatric, functional, and physiologic symptoms.Citation6 GBE is approved in Germany for the treatment of cerebral insufficiencyCitation10 and in China for the treatment of acute ischemic stroke.Citation11 Accumulating evidence of our group as well as investigators at other research institutes indicates that diterpene ginkgolides (DGs), which in this case are primarily ginkgolides A, B, C, and K, are responsible for the main pharmacological neuroprotection provided by GBE.Citation6,Citation12–Citation17
Neuroprotection is an important auxiliary treatment for improving the prognosis of patients with cerebral infarction.Citation3,Citation18 Given the aforementioned effects of rtPA and GBE, we hypothesized that the combination therapy of rtPA and GBE would not only generate a thrombolytic effect but also protect against the neurotoxicity of rtPA in patients with ischemic stroke.
Quantitatively analyzing the corresponding variations in biochemical metabolites using metabolomics methods has been shown to reveal drug action mechanisms.Citation19,Citation20 Unbiased overall metabolic profiling may shed new light on the molecular mechanisms targeted by both established and experimental pharmacotherapies,Citation21–Citation24 thereby facilitating the development of novel thrombolytic treatments that target defined metabolic pathways.
The prefrontal cortex (PFC) is a pivotal neuroanatomical hub with roles in various cognitive functions, including cognitive control and decision making.Citation25,Citation26 Moreover, the PFC has been shown to be involved in psychiatric disorders. For example, our previous studies have revealed that amino acid metabolic disturbances occur in the PFC in rat models of depression.Citation24,Citation27,Citation28
Taking together the foregoing evidence, in the present study, we investigated whether a single administration of rtPA led to neurotoxicity in normal rats and whether GBE or DG provided protective effects against any rtPA-induced neurotoxicity in the PFC of rats by examining the overall PFC metabolic profiles.
Materials and methods
Animals and experimental procedures
Male Sprague-Dawley (SD) rats (Chongqing Medical University, Chongqing, China) ~8 weeks old and weighing 230–250 g on the day of arrival were used for the experiments. Animals were group housed (5 rats/cage) and maintained with free access to food and water. All animal experiments were reviewed and approved by the Ethics Committee of Chongqing Medical University (permit number: 20120126). The experimental procedures were conducted abiding by the National Institutes of Health’s Guide for the Care and Use of Laboratory Animals.Citation29 Forty-five SD rats were randomly divided into the following four groups (n=15 rats in each group): 1) CON (saline administered through a caudal vein injection, CVI); 2) rtPA (10 mg/kg, CVI); 3) rtPA+DG (10 mg/kg plus 6.75 mg/kg, CVI), DG injected simultaneously with rtPA; and 4) rtPA+GBE (10 mg/kg plus 8 mg/kg, CVI), GBE injected simultaneously with rtPA. RtPA was provided by Boehringer Ingelheim Pharma GmbH & Co. KG (Ingelheim, Germany). Ginaton, a standardized gingko extract, was purchased from Dr Willmar Schwabe (Karlsruhe, Germany). DG meglumine injection acted as DG was provided by Jiangsu Kanion Pharmaceutical Co., Ltd. (Jiangsu, China). The doses used in this study were based on those used in previous studies.Citation16,Citation30–Citation32 The rats were sacrificed 24 hours after the injection by dislocation of the cervical vertebrae, and the whole brain was removed. The PFC was dissected, weighed, rapidly frozen with liquid nitrogen, and stored at −80°C until being used in assays.
Tissue sample pretreatment
To conduct gas chromatography–mass spectrometry (GC-MS) analyses, the PFC samples (40 mg) were transferred to a 1.5 mL centrifuge tube and submerged in 500 μL of a solution of water:methanol:chloroform (5:2:2, v/v/v). The mixture was sonicated for 5 minutes before being centrifuged at 14,000 rpm for 10 minutes. The supernatant (300 μL) was transferred to a glass sampling vial and vacuum dried at room temperature. The dried residue was dissolved in 80 μL of methoxamine hydrochloride (15 mg/mL pyridine) and incubated at 37°C for 90 minutes with continuous shaking. Then, 80 μL of bis(trimethylsilyl)trifluoroacetamide with 1% trimethyl chlorosilane was added, and the mixture was left to react for 1 hour at 70°C. The sample was derivatized and cooled to room temperature before being used in the assays.
GC–MS analysis
The GC-MS metabolomics procedure was conducted as described in our previous study.Citation33 Each aliquot (1 μL) of the derivative sample was injected in splitless mode into a LECO Pegasus 4D GCxGC-TOF mass spectrometer (LECO, San Jose, MI, USA). The components were separated on a DB-17MS column (30 m × 0.25 mm, inner diameter [I.D.]; J&W Scientific, Folsom, CA, USA) with high-purity helium carrier gas at a flow rate of 1 mL/min. The injector temperature was set at 280°C. The temperature of the electron ionization ion source was set at 220°C. The column temperature was initially kept at 60°C for 1 minute and then ramped from 60°C to 100°C at 8°C/min, ramped from 170°C to 210°C at 5°C/min, ramped from 210°C to 305°C at 15°C/min, and then held at 305°C for 7 minutes. MS spectra were acquired from m/z 30 to 600.
The chromatographic peaks in the total ion current (TIC) chromatograms represent corresponding metabolites, and their relative concentrations can be detected using the peak area normalization method. In the present study, the TIC chromatograms from the four PFC sample groups revealed strong signals for analysis, large peak capacity, and good retention time (RT) reproducibility. Individual peaks were detected in each group. These peaks were annotated by comparing the accurate mass (m/z) and RT from National Institute of Standards and Technology online databases. The relative intensities of these metabolites were used in the subsequent multivariate statistical analysis.
Data analysis
GC-MS-based metabolomics data were converted into network common data form (NetCdf) file format using Tag-Finder preprocessing software.Citation34 The subsequent processing information, containing peak indexes (RT-m/z), sample names, and normalized peak intensities, was imported into the SIMCA 11.5 software package (Umetrics, Umeå, Sweden). We used a principal component analysis (PCA) of quality control (QC) samples to evaluate the accuracy and repeatability of the GC-MS method (). PCA score plot demonstrated that the QC samples are clustered together. This showed that our GC-MS analysis method was robust and reliable. Multivariate statistical analyses, a supervised partial least squares discriminant analysis (PLS-DA) with Pareto scaling spectral data, was used to distinguish the difference between the groups of metabolic profiles. The R2Y and Q2 values were used to quantify the quality of the built model. A 200-iteration permutation test was conducted to avoid overfitting the model. A significant metabolite was denoted with a variable importance in the projection (VIP) score >1 and a P-value <0.05 (obtained from two-tailed Student’s t-test). A Venn diagram was calculated and placed on the website located at http://bioinformatics.psb.ugent.be/webtools/Venn/. Cytoscape software 3.4.0 was used to build the correlation network between these differential metabolites and the paired comparisons. GraphPad Prism 7.0 (GraphPad Software, Inc., La Jolla, CA, USA) was used for plotting the data.
Metabolic functions and pathway analysis
The module supporting pathway analysis on the MetaboAnalyst 3.0 website was used to identify the metabolic pathways significantly affected by these metabolites.Citation35 MetaboAnalyst was used to create a heat map of all the different metabolites, and Microsoft Visio 2013 was used to draw the schematic diagram. Ingenuity Pathway Analysis (IPA) Software (Qiagen, Redwood City, CA, USA) was used to analyze the different metabolites to obtain information about predicted molecular pathways and biological functions as described in http://www.ingenuity.com. IPA molecule activity predictor was used to predict the activity of molecules that were highly connected to these networks.
Results
PLS-DA model of GC-MS metabolomic analysis
PLS-DA score plot of CON, rtPA, rtPA+DG, and rtPA+GBE rats demonstrated clear discriminations among the four groups (R2X =0.693, R2Y =0.602, Q2 =0.46; ). Then, we used the metabolites from the pairwise groups (15 rtPA rats vs 15 CON rats, 15 rtPA+DG rats vs 15 rtPA rats, and 15 rtPA+GBE rats vs 15 rtPA rats) to build PLS-DA models. The pairwise PLS-DA score plots demonstrated clear discriminations between the rtPA and CON groups (R2X =0.595, R2Y =0.688, Q2 =0.564; ), the rtPA+DG and rtPA groups (R2X =0.666, R2Y =0.915, Q2 =0.761; ), and the rtPA+GBE and rtPA groups (R2X =0.717, R2Y =0.957, Q2 =0.596; ). The results of the 200-iteration permutation test showed that no overfitting had occurred; the models were valid and not overfit ().
Figure 1 Metabolomic analysis of PFC samples from CON, rtPA, rtPA+DG, and rtPA+GBE rats. (A) PLS-DA score plot for the comparison of CON, rtPA, rtPA+DG, and rtPA+GBE rats, (B) PLS-DA score plots for pairwise comparisons between CON and rtPA, (C) rtPA and rtPA+DG, or (D) rtPA and rtPA+GBE. (E) Statistical validation of the PLS-DA model by permutation testing of CON, rtPA, rtPA+DG, and rtPA+GBE rats, statistical validation of the PLS-DA model by permutation testing between (F) CON and rtPA, (G) rtPA and rtPA+DG, or (H) rtPA and rtPA+GBE. The criterion used for determination of validity was that all blue Q2 values to the left were lower than the original points to the right.
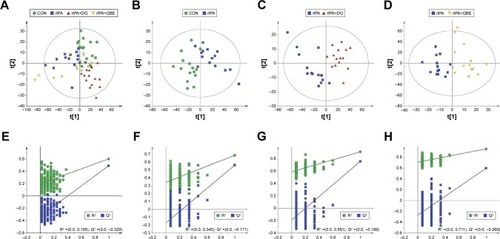
Identification of differential metabolites among the four groups
Twenty differential metabolites (VIP >1.0 and P<0.05) were identified between the rtPA and CON groups. As compared with the PFC samples in the CON group, those in the rtPA group demonstrated lower levels of 2,3-dihydroxypyridine, aminooxyacetic acid, N-ethylglycine, hexachlorobenzene, palmitic acid, N-carbamylglutamate, glycine, and phosphate, but higher levels of pyroglutamic acid, myoinositol, glutamic acid, aspartic acid, N-acetyl-l-aspartic acid (NAA), lactic acid, inosine, gamma-aminobutyric acid (GABA), N-acetyl-beta-alanine, glutamine, O-phosphoethanolamine, and glucose 6-phosphate (; and ).
Figure 2 Heat map of the differential metabolites in the rat PFC for comparisons of rtPA vs CON, rtPA+DG vs rtPA, and rtPA+GBE vs rtPA.
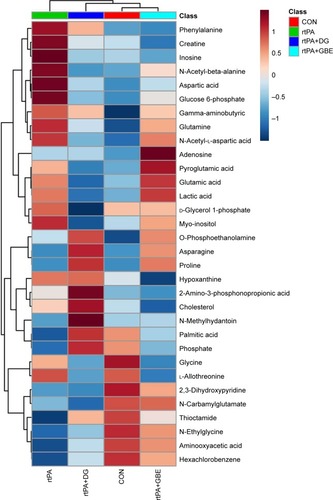
Figure 3 (A) Venn diagram of the differential metabolites in rat PFC for the comparisons of rtPA versus CON, rtPA+DG versus rtPA, and rtPA+GBE versus rtPA. (B) Correlation network between these differential metabolites and the three comparisons. The line colors represent the direction of the fold change in the differential metabolites. Red lines indicate that levels are upregulated in the comparison, whereas green boxes indicate the levels are downregulated. The line widths represent the VIP scores for the differential metabolites.
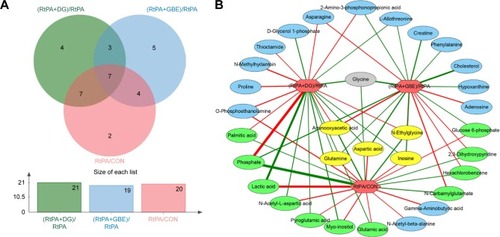
Table 1 Differential metabolites among the comparisons of rtPA/CON, rtPA+DG/rtPA, and rtPA+GBE/rtPA
A total of 21 differential metabolites were identified between the rtPA+DG and rtPA groups. As compared with those in the rtPA group, 10 metabolites were upregulated, and 11 metabolites were downregulated in the PFC samples derived from the rtPA+DG group (; and ). In comparing the rtPA+GBE and rtPA groups, 19 differential metabolites were identified. As compared with the PFC samples in the rtPA group, 10 metabolites were upregulated, and 11 metabolites were downregulated in samples from the rtPA+DG group (; and ).
There were 14 overlapping differential metabolites in the comparison sets of (rtPA+DG)/rtPA and rtPA/CON, among which 12 exhibited changes in the opposite direction, whereas the changes in the levels of O-phosphoethanolamine and glycine were in the same direction (). We found 11 overlapping differential metabolites in the comparison sets of (rtPA+GBE)/rtPA and rtPA/CON, among which 9 exhibited changes in the opposite direction, whereas changes in the levels of phosphate and glycine were in the same direction (). Among these metabolites, the changes in the levels of five core differential metabolites (ie, aminooxyacetic acid, N-ethylglycine, aspartic acid, inosine, and glutamine) in the comparison sets of (rtPA+DG)/rtPA and (rtPA+GBE)/rtPA were in the opposite direction with respect to those in the comparison set of rtPA/CON (). Glycine was the only core differential metabolite that exhibited changes in the same direction across the three comparison sets ().
In order to evaluate the effects of DG and GBE, we also made two paired comparisons of rtPA+DG versus CON and rtPA+GBE versus CON. The pairwise PLS-DA score plots demonstrated clear discriminations (). There were 20 differential metabolites found in the comparisons of rtPA+DG and CON (; ), among which the changes of aminooxyacetic acid, glutamine, N-ethylglycine and phosphate in the comparison sets of (rtPA+DG)/CON and rtPA/CON exhibited opposite direction with respect to those in the comparison set of (rtPA+DG)/rtPA. The results indicated that DG could ameliorate the metabolic disturbances of aminooxyacetic acid, glutamine, N-ethylglycine, and phosphate caused by rtPA. There were 8 differential metabolites (lactic acid, inosine, glutamic acid, myo-inositol, NAA, aspartic acid, pyroglutamic acid, and palmitic acid) found both in the comparison sets of (rtPA+DG)/rtPA and rtPA/CON, but not in the comparison sets of (rtPA+DG)/CON. The results indicated that DG could revise the metabolic disturbances of the 8 differential metabolites caused by rtPA. Meanwhile, there were 17 differential metabolites found in the comparisons of rtPA+GBE and CON (; ), among which GBE could ameliorate the metabolic disturbances of glutamine and revise the metabolic disturbances of the 8 differential metabolites (2,3-dihydroxypyridine, aminooxyacetic acid, N-carbamylglutamate, hexachlorobenzene, N-ethylglycine, glucose 6-phosphate, inosine, and aspartic acid) caused by rtPA.
Figure 4 PLS-DA score plots for pairwise comparisons between (A) CON and rtPA+DG or (B) CON and rtPA+GBE. Statistical validation of the PLS-DA model by permutation testing between (C) CON and rtPA+DG or (D) CON and rtPA+GBE. The criterion used for determination of validity was that all blue Q2 values to the left were lower than the original points to the right.
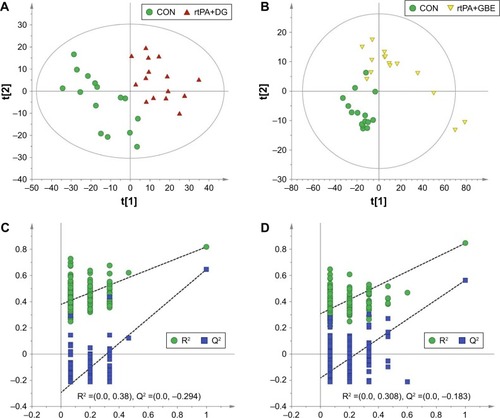
Figure 5 (A) Correlation network between these differential metabolites and the three comparisons of rtPA versus CON, rtPA+DG versus rtPA, and rtPA+DG versus CON. (B) Correlation network between these differential metabolites and the three comparisons of rtPA versus CON, rtPA+GBE versus rtPA, and rtPA+GBE versus CON.
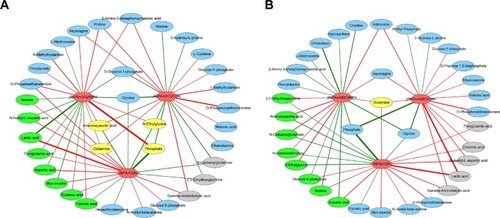
MetaboAnalyst pathway analysis of the differential metabolites
MetaboAnalyst 3.0 was used to identify the affected metabolic pathways based on the Kyoto Encyclopedia of Genes and Genomes metabolic library. According to the criteria of a false discovery rate <0.05 and an impact value >0, the comparison of rtPA versus CON showed that the metabolomic alterations were enriched in the pathways of “alanine, aspartate, and glutamate metabolism,” “D-glutamine and D-glutamate metabolism,” and “arginine and proline metabolism” (). The results of the rtPA+DG versus rtPA comparison showed that the metabolomic alterations were also enriched in the same pathways (). By contrast, no affected metabolic pathway was found in the comparison of rtPA+GBE versus rtPA. These three pathways mainly consist of asparagine, aspartic acid, creatine, GABA, glutamic acid, glutamine, NAA, and proline. The relative intensities of the differential metabolites in the four groups are shown in , and the results indicated that DG ameliorated the rtPA-induced metabolic disturbances through the “alanine, aspartate and glutamate metabolism,” “D-glutamine and D-glutamate metabolism,” and “arginine and proline metabolism” pathways. We generated a schematic model depicting the potentially disturbed metabolic pathways based on the MetaboAnalyst analysis of the functional roles and pathways of all the differential metabolites detected among the three comparisons ().
Figure 6 (A) Significant pathways of rtPA treatment-related differential metabolites. (B) Significant pathways of differential metabolites in the comparison set of rtPA+DG versus rtPA. (C) The relative intensity of the differential metabolites in the four groups. *P<0.05, **P<0.01, ***P<0.001.
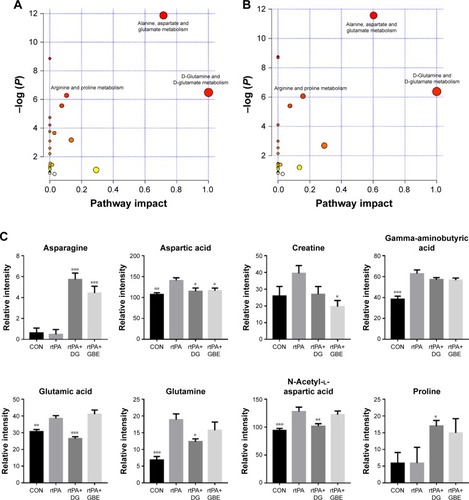
Figure 7 Simplified schematic diagram of the metabolic changes induced by administration of rtPA, DG, and GBE. Red boxes indicate upregulation in the comparison, yellow boxes indicate no change, and green boxes indicate downregulation.
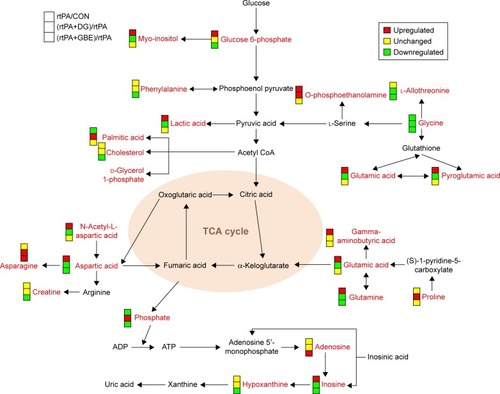
Discussion
Previous studies have revealed that in addition to thrombolysis, rtPA administration may cause adverse neurological effects, which may lead to clinical exacerbation in stroke patients.Citation3,Citation31,Citation36 Administration of rtPA can induce not only angioedema through blood–brain barrier disruption but also cytotoxic brain edema through excitotoxic neurotoxicity.Citation37 Therefore, a detailed understanding of the specific neurotoxicity mechanisms for rtPA and strategies to prevent the toxicity induced by treatment with rtPA are needed. Because GBE and DG have been suggested to have many beneficial effects on CNS function and are widely used for the treatment of cerebral insufficiency and acute ischemic stroke,Citation10,Citation11 we hypothesized that combining GBE or DG with rtPA would increase the efficacy of rtPA and protect against rtPA-induced neurotoxicity in patients with ischemic stroke.
In this study, we used GC-MS metabolomic analyses to demonstrate, for the first time, that a single administration of rtPA led to metabolic disturbances in the rat PFC. We also used this analysis following administration of rtPA combined with GBE or DG and found that the metabolic signature of the PFC in the rtPA group was significantly distinguished from that in the CON, rtPA+DG, or rtPA+GBE groups. A total of 32 differential metabolites in rat PFC were altered among the three paired comparisons of rtPA versus CON, rtPA+DG versus rtPA, and rtPA+GBE versus rtPA. To develop a deeper insight into the underlying molecular mechanisms of rtPA, DG, and GBE, we conducted a functional analysis and determined that these identified 32 differential metabolites were primarily involved in 1) neurotransmitter metabolism, 2) amino acid metabolism, 3) energy metabolism, 4) lipid metabolism, and 5) nucleotide metabolism.
Neurotransmitter metabolism
The most significant alterations in the comparative metabolomic analysis of rtPA and CON were attributed to changes in neurotransmitter metabolism, primarily glutamate and aspartate metabolism. Our results showed that rtPA upregulated the production of glutamic acid, aspartic acid, NAA, GABA, and glutamine but downregulated the production of glycine. Although both DG and GBE ameliorated the upregulation of aspartic acid and glutamine, DG also ameliorated the upregulation of glutamic acid and NAA. Neither DG nor GBE ameliorated the rtPA-induced changes in GABA and glycine.
As excitatory amino acid neurotransmitters, glutamic acid and aspartic acid are known to be neurotoxic in cell cultureCitation38,Citation39 and to cause local neuronal death.Citation40,Citation41 Glutamic acid can be converted into glutamine when catalyzed by glutamine synthetase.Citation42 There is a direct evidence showing that glutamine induces mitochondrial permeability transitionCitation43 and production of free radicalsCitation44 in cultured astrocytes. GABA is the primary inhibitory neurotransmitter in the CNS. Disturbances in the GABA system have been linked to neuropsychiatric disorders, for instance, PFC-dependent working memory deficits,Citation45 epilepsy,Citation46 and schizophrenia.Citation47
We also found reduced glycine levels in the rtPA/CON, rtPA+DG/rtPA, and rtPA+GBE/rtPA comparisons, indicating that both DG and GBE strengthened the rtPA-induced reduction of glycine levels. Glycine can markedly potentiate NMDA-mediated neurotoxicity.Citation48,Citation49 However, according to the IPA analysis, the changes in the overall differential metabolites of rtPA and CON lead to the activation of NMDA receptor (). Although rtPA was used in combination with GBE, the treatment could still lead to less activation of NMDA receptor (). It is worth noting that the treatment of rtPA combined with DG could lead to inhibition of NMDA receptor (). Thus, based on our findings, both DG and GBE may exert the neuroprotective effect by reducing the activation of NMDA receptor caused by rtPA.
Figure 8 Network of “amino acid metabolism, cell-to-cell signaling and interaction, molecular transport” associated with key differential metabolites in the comparison of rtPA and CON groups in rats PFC with a score of 38 and 14 differential metabolites involved.
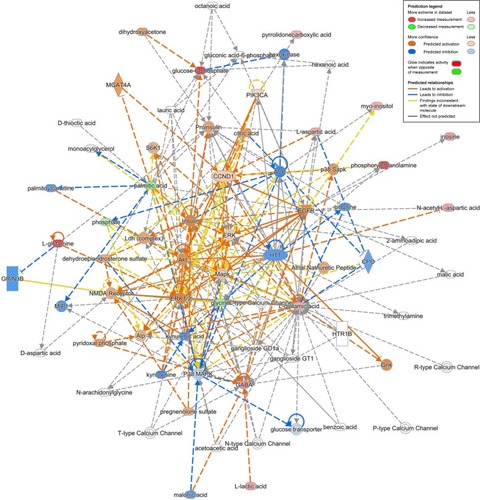
Figure 9 Network of “amino acid metabolism, cell-to-cell signaling and interaction, molecular transport” associated with key differential metabolites in the comparison of rtPA+GBE and CON groups in rats PFC with a score of 40 and 15 differential metabolites involved.
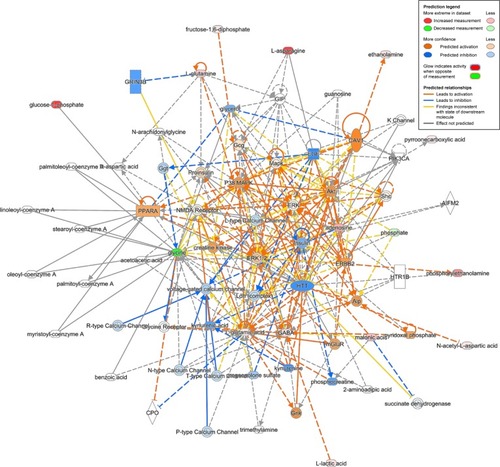
Figure 10 Network of “cellular compromise, lipid metabolism, small molecule biochemistry” associated with key differential metabolites in the comparison of rtPA+DG and CON groups in rats PFC with a score of 38 and 14 differential metabolites involved.
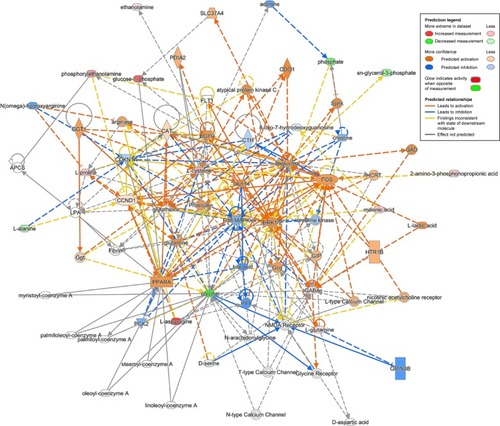
Based on our metabolomics results and pathway analysis, DG may exert its neuroprotective effects by reducing the excess production of glutamate and aspartate excitotoxicity and revising the activation of NMDA receptor induced by rtPA in the PFC of rats, while GBE may partially ameliorate the excitotoxicity induced by rtPA.
Amino acid metabolism
Our results demonstrated an elevation in N-acetyl-beta-alanine and pyroglutamic acid and a reduction in aminooxyacetic acid and N-carbamylglutamate levels in the rtPA group compared with those in the CON group. DG ameliorated the rtPA-induced upregulation of pyroglutamic acid and aminooxyacetic acid, whereas GBE ameliorated the downregulation of aminooxyacetic acid and N-carbamylglutamate. Aminooxyacetic acid, a core metabolite, has been reported to increase the level of GABA in rat brain by inhibiting 4-aminobutyrate aminotransferase activity.Citation50 Aminooxyacetic acid also has anticonvulsant properties and can reduce focal cerebral ischemia injury.Citation51,Citation52 The schematic diagram () shows that while pyroglutamic acid is the downstream metabolite of glutathione, glycine is the upstream metabolite. Thus, the downregulation of glycine and the upregulation of pyroglutamic acid reflect the rtPA-induced disturbances in glutathione metabolism. We found that DG ameliorated the upregulation of pyroglutamic acid. Administration of rtPA downregulated N-carbamylglutamate, a drug derivative of N-acetylglutamate that activates the first enzyme in the urea cycleCitation53 but has not been reported in the CNS. This downregulation was ameliorated by GBE.
Energy metabolism
Our results demonstrated a lactic acid level elevation in the rtPA versus CON group analysis and reduction in the rtPA+DG versus rtPA group comparison analysis. The accumulation of lactic acid has been implicated in the deaths of both neurons and glia.Citation54 Thus, DG may protect normal cells against demise in the PFC. In the glycolysis pathway, glucose-6-phosphate and myo-inositol were found to be elevated in the rtPA versus CON group analysis. DG ameliorated the upregulation of myo-inositol and glucose-6-phosphate induced by rtPA. However, administration of rtPA or DG did not alter metabolites in the tricarboxylic acid (TCA) cycle. Thus, our results indicated that rtPA, DG, and GBE exert only limited influence on glycolysis and the TCA cycle.
Lipid metabolism
Four metabolites belonging to lipid metabolism (palmitic acid, O-phosphoethanolamine, cholesterol, and D-glycerol 1-phosphate) were differentially altered in the three paired comparisons. However, only palmitic acid and O-phosphoethanolamine were affected by the treatment with rtPA. Palmitic acid has been found to modify the cysteine residues of proteolipid protein (PLP) to form thioester linkages.Citation55 Based on our findings, DG may restore the rtPA-induced reduced level of palmitic acid in the PFC, and this would facilitate the synthesis of PLP, which participates in the formation of myelin. We also found that DG enhanced the rtPA-induced increase in O-phosphoethanolamine in the PFC.
Nucleotide metabolism
Four metabolites belonging to nucleotide metabolism (phosphate, inosine, adenosine, and hypoxanthine) were differentially altered in the three paired comparisons. A reduction of inosine was observed in both the rtPA+DG/rtPA and rtPA+GBE/rtPA comparisons, but an increase was found in the rtPA/CON comparison. Inosine can inhibit platelet activation and reduce cerebral infarction.Citation56,Citation57 Here, the strengthening effects of thrombolysis caused by inosine may potentially become a target for reducing cerebral hemorrhage risk by using DG or GBE combined with rtPA. Phosphate is a critically important molecule in various reactions. Our results revealed that DG, but not GBE, restored the reduced level of phosphate caused by rtPA in the PFC. However, GBE upregulated the production of adenosine, which acts as a neuromodulator and a homeostatic modulator in the CNS.Citation58
Others
There were six other metabolites (2,3-dihydroxypyridine, hexachlorobenzene, thioctamide, 2-amino-3-phosphonopropionic acid, N-methylhydantoin, and N-ethylglycine) among the three paired comparisons that were differentially altered, but these metabolites could not be definitively classified. Among these, our results demonstrated that N-ethylglycine was elevated by the rtPA administration and that this elevation was ameliorated by both DG and GBE. This metabolite reportedly shows antinociceptive effects in experimental inflammatory and neuropathic pain.
Based on the results of the above categories of functions analysis, the molecular mechanisms of rtPA, DG, and GBE mainly involved in neurotransmitter, amino acid, energy, lipid, and nucleotide metabolism. Neuroprotective effects of DG are mainly related to glutamate, aspartate, and nucleotide metabolism pathways and revising the activation of NMDA receptor induced by rtPA. However, the neuroprotective effects of GBE are only related to aspartate and nucleotide metabolism pathways. GBE may partially ameliorate the excitotoxicity induced by rtPA. GBE is a mixture of substances with many different physical and chemical properties. Numerous pharmacological investigations have led to the conclusion that DG is responsible for the major pharmacological neuroprotective effects. Our results also confirmed the conclusion that DG demonstrated better effects than GBE.
Limitations
There were several limitations in our study that should be acknowledged. Our experiments were conducted with male, normal, non-pathological rats to more clearly reveal the neurotoxicity of rtPA; however, the use of a rat model of ischemia may help us to better understand the neuroprotective effects of DG and GBE and to detect the thrombolytic effect of rtPA combined with DG or GBE and comparing male and female rats in future studies which would have helped to ascertain if there are gender differences in the effects of tPA, DG, and GBE. There were no analyses of a single dose of DG and GBE in this study. To better elaborate the neuroprotective mechanism of DG and GBE, additional metabolomic analysis should be employed in future studies. In addition, our experiments were conducted on samples derived from the PFC, but peripheral metabolic effects and primary cell experiments in vitro may help us to better understand the neurotoxicity of rtPA and the neuroprotective effects of DG and GBE.
Conclusion
Our comparative metabolomics results provided the novel finding that rtPA may cause metabolic disturbances in the PFC of adult rats. Both GBE and its constituent DGs effectively attenuated the rtPA-induced disturbances in neurotransmitter, amino acid, energy, lipid, and nucleotide metabolisms. Compared with GBE, DG better controlled the glutamate and aspartate excitotoxicity and the activation of NMDA receptor induced by rtPA. Through investigating the PFC metabolic profiles following a single administration of rtPA or of rtPA combined with DG or GBE, this study has offered important novel mechanistic insights into the adverse effects induced by rtPA and provided directions for future exploration on the thrombolytic effects of rtPA combined with DG or GBE in humans.
Author contributions
All authors contributed toward data analysis, drafting and revising the paper and agree to be accountable for all aspects of the work.
Acknowledgments
This work was supported by the National Basic Research Program of China (973 Program, grant no. 2009CB918300), National Key R&D Program of China (grant no. 2017YFA0505700), the National Natural Science Foundation of China (grant nos. 81371310, 81701360), the Natural Science Foundation of Inner Mongolia Autonomous Region of China (grant no. 2016MS0884), the Foundation Project of the Inner Mongolia Autonomous Region People’s Hospital (grant no. 201551), and the China Postdoctoral Science Foundation Funded Project (project no. 2015M570772).
Supplementary materials
Figure S1 Principal component analysis (PCA) score plot of all samples. R2X =0.987, Q2 =0.859. The main parameters of PCA are the two principal components, including R2X and Q2. Generally, the main parameter for judging the quality of the model is R2X, which indicates how well the model explains the data. A value of 0.4 indicates that the model is reliable.
Abbreviations: rtPA, recombinant tissue plasminogen activator; DG, diterpene ginkgolide; GBE, Ginkgo biloba extract; QC, quality control; CON, control.
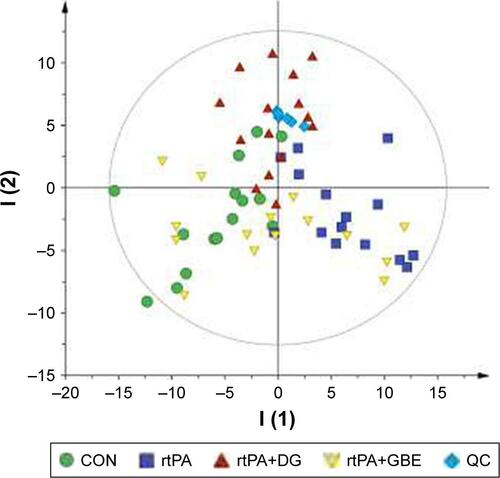
Table S1 Key differential metabolites in the comparison of rtPA+DG and CON groups in rat PFC
Table S2 Key differential metabolites in the comparison of rtPA+GBE and CON groups in rat PFC
Disclosure
The authors report no conflicts of interest in this work.
References
- EmbersonJLeesKRLydenPEffect of treatment delay, age, and stroke severity on the effects of intravenous thrombolysis with alteplase for acute ischaemic stroke: a meta-analysis of individual patient data from randomised trialsLancet201438499581929193525106063
- HackeWKasteMBluhmkiEThrombolysis with alteplase 3 to 4.5 hours after acute ischemic strokeN Engl J Med2008359131317132918815396
- DongMXHuQCShenPRecombinant tissue plasminogen activator induces neurological side effects independent on thrombolysis in mechanical animal models of focal cerebral infarction: a systematic review and meta-analysisPLoS One2016117e015884827387385
- DongMXLiCMShenPRecombinant tissue plasminogen activator induces long-term anxiety-like behaviors via the ERK1/2-GAD1-GABA cascade in the hippocampus of a rat modelNeuropharmacology201712811928986280
- PanCLiuNZhangPEGb761 ameliorates neuronal apoptosis and promotes angiogenesis in experimental intracerebral hemorrhage via RSK1/GSK3beta pathwayMol Neurobiol20185521556156728185127
- MaclennanKMDarlingtonCLSmithPFThe CNS effects of Ginkgo biloba extracts and ginkgolide BProg Neurobiol200267323525712169298
- SnitzBEO’MearaESCarlsonMCGinkgo biloba for preventing cognitive decline in older adults: a randomized trialJAMA2009302242663267020040554
- BeekTAVGinkgolides and bilobalide: their physical, chromatographic and spectroscopic propertiesBioorg Med Chem200513175001501215993092
- PrehnJHKrieglsteinJPlatelet-activating factor antagonists reduce excitotoxic damage in cultured neurons from embryonic chick tel-encephalon and protect the rat hippocampus and neocortex from ischemic injury in vivoJ Neurosci Res19933421791888095559
- Le BarsPLKatzMMBermanNItilTMFreedmanAMSchatzbergAFA placebo-controlled, double-blind, randomized trial of an extract of Ginkgo biloba for dementia. North American EGb Study GroupJAMA199727816132713329343463
- ZengXLiuMYangYLiYAsplundKGinkgo biloba for acute ischaemic strokeCochrane Database Syst Rev20054CD003691
- BaiSZhangXChenZInsight into the metabolic mechanism of Diterpene Ginkgolides on antidepressant effects for attenuating behavioural deficits compared with venlafaxineSci Rep201771959128852120
- MaSLiuXXuQZhangXTransport of ginkgolides with different lipophilicities based on an hCMEC/D3 cell monolayer as a blood-brain barrier cell modelLife Sci201411429310125139831
- OberpichlerHSauerDRossbergCMennelHDKrieglsteinJPAF antagonist ginkgolide B reduces postischemic neuronal damage in rat brain hippocampusJ Cereb Blood Flow Metab19901011331352298830
- LiebgottTMiollanMBerchadskyYDrieuKCulcasiMPietriSComplementary cardioprotective effects of flavonoid metabolites and terpenoid constituents of Ginkgo biloba extract (EGb 761) during ischemia and reperfusionBasic Res Cardiol200095536837711099163
- LiangZBaiSShenPGC-MS-based metabolomic study on the antidepressant-like effects of diterpene ginkgolides in mouse hippocampusBehav Brain Res201631411612427498146
- GengJLAaJYFengSQExploring the neuroprotective effects of Ginkgolides injection in a rodent model of cerebral ischemia-reperfusion injury by GC-MS based metabolomic profilingJ Pharm Biomed Anal201714219028514718
- KikuchiKTanakaEMuraiYTancharoenSClinical trials in acute ischemic strokeCNS Drugs2014281092993825160686
- LindonJCNicholsonJKHolmesEContemporary issues in toxicology the role of metabonomics in toxicology and its evaluation by the COMET projectToxicol Appl Pharmacol2003187313714612662897
- NicholsonJKWilsonIDUnderstanding “global” systems biology: metabonomics and the continuum of metabolismNat Rev Drug Discov20032866867612904817
- BaiSZhouCChengP1H NMR-based metabolic profiling reveals the effects of fluoxetine on lipid and amino acid metabolism in astrocytesInt J Mol Sci20151648490850425884334
- ZhengPWangYChenLIdentification and validation of urinary metabolite biomarkers for major depressive disorderMol Cell Proteomics201312120721423111923
- LiuLZhouXZhangYThe identification of metabolic disturbances in the prefrontal cortex of the chronic restraint stress rat model of depressionBehav Brain Res201630514815626947756
- ChenGYangDYangYAmino acid metabolic dysfunction revealed in the prefrontal cortex of a rat model of depressionBehav Brain Res201527828629224861712
- GläscherJAdolphsRDamasioHLesion mapping of cognitive control and value-based decision making in the prefrontal cortexProc Natl Acad Sci U S A201210936146811468622908286
- WardenMRSelimbeyogluAMirzabekovJJA prefrontal cortex-brainstem neuronal projection that controls response to behavioural challengeNature2012492742942843223160494
- BaiSHuQChenZBrain region-specific metabolite networks regulate antidepressant effects of venlafaxineRSC Adv20177734635846369
- ZhengPZengBZhouCGut microbiome remodeling induces depressive-like behaviors through a pathway mediated by the host’s metabolismMol Psychiatry201621678679627067014
- ClarkJDGebhartGFGonderJCKeelingMEKohnDFThe 1996 guide for the care and use of laboratory animalsILAR J1997381414811528046
- LapergueBDangBQDesillesJPHigh-density lipoprotein-based therapy reduces the hemorrhagic complications associated with tissue plasminogen activator treatment in experimental strokeStroke201344369970723422087
- ZuoWChenJZhangSIMM-H004 prevents toxicity induced by delayed treatment of tPA in a rat model of focal cerebral ischemia involving PKA-and PI3K-dependent Akt activationEur J Neurosci20143912210724649933
- WangYPeiDSJiHXXingSHProtective effect of a standardized Ginkgo extract (ginaton) on renal ischemia/reperfusion injury via suppressing the activation of JNK signal pathwayPhytomedicine2008151192393118929474
- LiJTangGChengKPeripheral blood mononuclear cell-based metabolomic profiling of a chronic unpredictable mild stress rat model of depressionMol Biosyst201410112994300125182291
- LuedemannAStrassburgKErbanAKopkaJTagFinder for the quantitative analysis of gas chromatography–mass spectrometry (GC-MS)-based metabolite profiling experimentsBioinformatics200824573273718204057
- XiaJWishartDSUsing MetaboAnalyst 3.0 for comprehensive metabolomics data analysisCurr Protoc Bioinformatics20165514 10 1114 10 91
- YepesMSandkvistMMooreEGBuggeTHStricklandDKLawrenceDATissue-type plasminogen activator induces opening of the blood-brain barrier via the LDL receptor-related proteinJ Clin Invest2003112101533154014617754
- YepesMRousselBDAliCVivienDTissue-type plasminogen activator in the ischemic brain: more than a thrombolyticTrends Neurosci2009321485518963068
- RothmanSMOlneyJWGlutamate and the pathophysiology of hypoxic–ischemic brain damageAnn Neurol19861921051112421636
- LauATymianskiMGlutamate receptors, neurotoxicity and neurodegenerationPflügers Arch2010460252554220229265
- OlneyJWNeurotoxicity of excitatory amino acidsKainic Acid As A Tool in Neurobiology197895121
- ManganoRMSchwarczRChronic infusion of endogenous excitatory amino acids into rat striatum and hippocampusBrain Res Bull198310147516130829
- AlbrechtJNorenbergMDGlutamine: a Trojan horse in ammonia neurotoxicityHepatology200644478879417006913
- RaoKRJayakumarANorenbergMInduction of the mitochondrial permeability transition in cultured astrocytes by glutamineNeurochem Int200343451752312742099
- JayakumarARRama RaoKSchousboeANorenbergMDGlutamine-induced free radical production in cultured astrocytesGlia200446329630115048852
- McQuailJAFrazierCJBizonJLMolecular aspects of age-related cognitive decline: the role of GABA signalingTrends Mol Med201521745046026070271
- BradfordHGlutamate, GABA and epilepsyProg Neurobiol19954764775118787032
- TaylorSFTsoIFGABA abnormalities in schizophrenia: a methodological review of in vivo studiesSchizophr Res20151671–3849025458856
- McNamaraDDingledineRDual effect of glycine on NMDA-induced neurotoxicity in rat cortical culturesJ Neurosci19901012397039761980134
- PatelJZinkandWCThompsonCKeithRSalamaARole of glycine in the N-methyl-d-aspartate-mediated neuronal cytotoxicityJ Neurochem19905438498542106010
- CarmonaEGomesCTrolinGEffect of aminooxyacetic acid (AOAA) on GABA levels in some parts of the rat brainNaunyn Schmiedebergs Arch Pharmacol1980312151557393347
- DavanzoJPGreigMECroninMAAnticonvulsant properties of amino-oxyacetic acidAm J Physiol196120183383713883717
- LiG-FZhangJ-XLuoH-KEffect of aminooxyacetic acid on focal cerebral ischemia injury in ratsChin J Anesthesiol2011318984986
- DaniottiMLaMGFioriniPFilippiLNew developments in the treatment of hyperammonemia: emerging use of carglumic acidInt J Gen Med20114212821403788
- NedergaardMGoldmanSADesaiSPulsinelliWAAcid-induced death in neurons and gliaJ Neurosci1991118248924971869926
- WeimbsTStoffelWProteolipid protein (PLP) of CNS myelin: positions of free, disulfide-bonded, and fatty acid thioester-linked cysteine residues and implications for the membrane topology of PLPBiochemistry1992314912289122961281423
- HsiaoGLinKHChangYProtective mechanisms of inosine in platelet activation and cerebral ischemic damageArterioscler Thromb Vasc Biol20052591998200415976325
- ShenHChenG-JHarveyBKBickfordPCWangYInosine reduces ischemic brain injury in ratsStroke200536365465915692110
- GomesCVKasterMPToméARAgostinhoPMCunhaRAAdenosine receptors and brain diseases: neuroprotection and neurodegenerationBiochim Biophys Acta2011180851380139921145878