Abstract
The final goal in magnetic hyperthermia research is to use nanoparticles in the form of a colloidal suspension injected into human beings for a therapeutic application. Therefore the challenge is not only to develop magnetic nanoparticles with good heating capacities, but also with good colloidal properties, long blood circulation time and with grafted ligands able to facilitate their specific internalisation in tumour cells. Significant advances have been achieved optimising the properties of the magnetic nanoparticles, showing extremely large specific absorption rate values that will contribute to a reduction in the concentration of the magnetic fluid that needs to be administered. In this review we show the effect of different characteristics of the magnetic particles, such as size, size distribution and shape, and the colloidal properties of their aqueous suspensions, such as hydrodynamic size and surface modification, on the heating capacity of the magnetic colloids.
Introduction
Recent studies have reported the development of theranostic magnetic nanomedicines capable of directing a drug toward a target tissue or cell, while allowing simultaneous monitoring of the response to the treatment [Citation1,Citation2,Citation3]. The advantage of this approach is the high drug loading on the nanoparticle surface due to their small size (i.e. their high specific surface), and the ability to magnetically address it to the tumour site that limits the biodistribution and hence reduces any adverse effects. The therapeutic effect of the drug can be enhanced if magnetic nanoparticles accumulated in a specific area are heated above body temperature under the action of an alternating magnetic field (magnetothermia or magnetic hyperthermia). The heating efficiency depends on the magnetic properties of the nanoparticles, the strength and frequency of the magnetic field, and the cooling capacity of the blood flow in the target site [Citation4,Citation5]. Furthermore, nanoparticles can be loaded with specific antibodies to facilitate tumour targeting [Citation6].
Encouraging preclinical data of hyperthermia treatments have been obtained in cancer patients [Citation7] and numerous reviews have been published on the subject [Citation8–11]. The treatment still requires some improvements related to the selective heat induction in tumour sites, the homogeneity of the temperature distribution into the tumour [Citation12,Citation13], and the reduction of the invasiveness of the technique. In cancer therapy, it seems that the future of the theranostic agents is likely to be employed in synergic combination with other therapies [Citation14]. For example, it has been reported that magnetic heating can enhance the effect of radiation or drugs [Citation7,Citation15,Citation16]. Nowadays, there is intense research to identify the best magnetic nanoparticles that with the minimum amount of material allows for sufficient and homogeneous heating within the appropriate field parameters.
Magnetic nanoparticles for biomedical applications must combine a handful of key features, from the colloidal to the magnetic properties, for optimal behaviour [Citation17]. Thus, particles should show small hydrodynamic size and narrow size distribution to ensure colloidal stability, long blood residence time and possibilities for crossing biological barriers such as cell junctures and membranes. Their surface coating must guarantee biocompatibility and, if possible, provide specificity for biological target sites. Finally, it is obvious that nanoparticles should exhibit good magnetic properties in terms of high magnetisation values and high magnetic susceptibility for an optimum performance in diagnosis and therapy. Nanoparticle size and shape are key features controlling the magnetic moment and the magnetisation rotation mechanism that governs how a material responds to an alternating magnetic field. The degree of crystallinity and the magnetic interactions between particles also have a strong influence on the magnetic properties. In most cases the synthesis is responsible for these material features, such as the presence of impurities or defects, and the formation of irreversible agglomeration, which are reflected in their magnetic properties.
To identify the most suitable material for this application, it is worth considering the main mechanisms of heat generation: 1) eddy currents, 2) hysteresis losses (more important for multi-domain particles) and 3) Néel and Brownian relaxations (for single-domain nanoparticles) [Citation10]. Those hyperthermia mechanisms have been extensively studied and are not the objective of this review. Nevertheless, it is useful to briefly consider their implications here. Thus, the heating efficiency will depend on the material properties as commented above and on the amplitude and frequency of the applied field. Therefore, key parameters inherent to the nanoparticles (in which this review will focus) are size and hydrodynamic size, size distribution, shape, crystallinity, chemical composition, concentration and other parameters affecting inter-particle interactions such as the viscosity of the medium. Key parameters not inherent to the nanomaterial are the amplitude and the frequency of the applied magnetic field. Appropriate field conditions depend on the properties of the material in such a way that, given two samples of nanoparticles A and B, there will be some field conditions for which the heating efficiency of A will be greater than that of B, while for other field conditions the result will be the opposite, B heating more than A [Citation18].
The advantage of working with superparamagnetic nanoparticles (below a certain size, ferro- and ferrimagnetic materials exhibit a thermally activated spin fluctuation behaviour) is that it is easier to keep their colloidal stability due to their smaller size, avoiding agglomeration and therefore the potential embolisation of capillary vessels. It has been shown that the presence of superparamagnetic particles leads to a powerful enhancement of proton relaxation times in nuclear magnetic resonance imaging (MRI) in contrast to ferromagnetic particles [Citation19,Citation20]. The effect of the nanoparticle size on the heating capacity of a suspension under an alternating magnetic field is not so clear [Citation21]. In principle, large particles (> 10 nm) are preferred due to their larger magnetic moment while keeping coherent magnetisation rotation (< 70 nm) [Citation3]. However, magnetic interactions between particles are stronger as particle size increases, leading to undesirable collective behaviours [Citation22,Citation23] and poor colloidal stability. Particle sizes larger than 10 nm and smaller than 50 nm seem to be ideal for this application.
Iron oxide-based nanoparticles are the most common materials in magnetic hyperthermia research [Citation24,Citation25] and chemical precipitation in water and thermal decomposition in organic media are by far the most commonly employed methods for the synthesis. Other methods, like the polyol process and electro-oxidation [Citation26,Citation27], have been reported to a lesser extent. The choice of the synthetic procedure is the first step when planning to apply magnetic nanoparticles for hyperthermia and it is a crucial selection that will determine the physicochemical properties of the magnetic nanoparticles (MNPs) and, therefore, their potential success for this application.
In this review we will focus on calorimetric measurements performed with liquid suspensions of MNPs to evaluate the heating capacity of a material. We will pay special attention to the effect of different characteristics of the magnetic particles such as size, size distribution and shape, and colloidal properties of aqueous suspensions, such as hydrodynamic size and surface modification. These physicochemical properties are related to the synthesis procedure and can severely affect the heating capacity of magnetic colloids. Other important features are the coating and the hydrodynamic size, which will also affect the cell uptake [Citation28]. It seems that hyperthermia treatment could be more effective if nanoparticles are internalised by the cells [Citation29], although the activation of cation channels in cells when the nanoparticles are bound to the plasma membrane has also been reported [Citation30]. The most common physical magnitude for reporting the calorific power of MNPs is the specific absorption rate (SAR), also called specific loss power (SLP). It is the energy absorption rate per gram of material during exposition to an alternating magnetic field and is calculated from the expression in Equation 1 [Citation10].
where ΔT is the increase in temperature, Δt is the measurement time, and c is the specific heat of the sample. Experimentally the ratio ΔT/Δt is the initial slope of the temperature versus time dependence [Citation31].
Comparison between published SAR values will be discussed although it is difficult due to the lack of any standardised protocols to characterise magneto-thermal properties.
Synthesis method
Interesting SAR values have been reported for MNPs larger than 10 nm. Aqueous synthesis methods such as the co-precipitation of Fe(II) and Fe(III) salts, described by Massart, and its modifications, provides MNPs with sizes between 3 and 12 nm. Precipitation in water of Fe(II) salts described by Sugimoto for the synthesis of MNPs allows preparation of particles with sizes ≥30 nm [Citation32]. This route has been explored for the preparation of particles suitable for hyperthermia, and the first results showed that the smaller particles (30–50 nm), within the theoretical monodomain range, are better than larger particles (>50 nm), which exhibited nearly null heating efficiency, in spite of their high saturation magnetisation (MS) values [Citation33,Citation34]. The differences may be due to the use of fields insufficient to saturate the largest particles. The main drawback of the Sugimoto route lies in the poor colloidal stability of the MNPs due to their large size and the absence of surfactants, complicating their use for biomedicine. For that reason, to obtain biocompatible and stable MNPs by this approach, the main challenges are to activate the surface and develop efficient coatings.
Thermal decomposition in organic media is a well-known method of preparing nanoparticles larger than 10 nm [Citation35]. Tuning the size of MNPs prepared by thermal decomposition is possible by adjusting experimental parameters such as the nature of the iron precursor (e.g. Fe(CO)5, Fe(acac)3, Fe(carboxylate)3, FeO(OH)), the nature of the stabilisers (e.g. long-chain carboxylic acids, long-chain amines, phosphines, alcohols), concentration of the reagents, temperature and temperature ramp or stirring rate [Citation36]. One strategy to increase nanoparticle size is the seed-growth process. This method, however, should be carefully used because it can lead to MNPs with crystal defects as has been recently reported [Citation37]. The nanoparticles were apparently highly crystalline, even when observed by high-resolution transmission electron microscopy (HRTEM), but a thorough strain tensors study revealed that in fact those MNPs had defects consisting in strained regions (). Those subtle defects were responsible for shifting down saturation magnetisation and SAR values dramatically.
Figure 1. HRTEM micrographs of nanoparticles obtained by thermal decomposition controlling the size by seed-growth (showing strains responsible for lowering the magnetisation. Reproduced with permission from Levy et al. [Citation37]).
![Figure 1. HRTEM micrographs of nanoparticles obtained by thermal decomposition controlling the size by seed-growth (showing strains responsible for lowering the magnetisation. Reproduced with permission from Levy et al. [Citation37]).](/cms/asset/66dd276a-beeb-4f38-8618-7e88c40bfcbb/ihyt_a_826824_f0001_b.jpg)
In another approach, highly uniform nanoparticles up to 22 nm were prepared with good properties for hyperthermia by a modification of the iron(III) oleate decomposition method described by Park et al. [Citation38]. In this case size control was achieved by changing surfactant concentration (oleic acid in octadecene) and reaction times to affect nucleation and growth rates () [Citation39]. Finally, the synthesis of nanoparticles up to 180 nm free of internal defects has also been achieved by thermal decomposition in organic media using decanoic acid as surfactant and benzyl ether as solvent and controlling the temperature ramp [Citation40,Citation41].
Figure 2. HRTEM and TEM micrographs of nanoparticles obtained by thermal decomposition, modifying surfactant concentration and reaction time (reproduced with permission of the Royal Society of Chemistry from Salas et al. [Citation39]).
![Figure 2. HRTEM and TEM micrographs of nanoparticles obtained by thermal decomposition, modifying surfactant concentration and reaction time (reproduced with permission of the Royal Society of Chemistry from Salas et al. [Citation39]).](/cms/asset/49a548e2-59b4-4afc-bb00-ffed6da45309/ihyt_a_826824_f0002_b.jpg)
Data concerning calorimetric experiments in the literature usually differ a lot in the experimental conditions, including the amplitude and the frequency of the applied alternating magnetic field. Nevertheless, it is possible to compare some works and to extract some important conclusions, always keeping in mind that the maximum SAR for a particle will depend on the field and frequency. shows data from samples synthesised by co-precipitation and thermal decomposition. Co-precipitation in water usually yields MNPs with broad size distributions, and consequently (as will be discussed below), low SAR values. However, when those MNPs are subjected to a size selection process, the heating efficiency under the same applied field is comparable to the one achieved by MNPs synthesised by thermal decomposition [Citation41,Citation42]. On the other hand, as commented before, if seed-growth is employed to increase the size of MNPs synthesised by thermal decomposition, SAR values drop dramatically [Citation37]. The crystallinity and structural defects that cause this are mainly related to the synthesis temperature and the presence of impurities.
Figure 3. (A) Comparison between the experimental SAR values (W/g Fe) of nanoparticles of different sizes obtained by three different synthetic approaches: 1) co-precipitation in water followed by a size selection process (□, 31 mT, 700 kHz) [Citation42], 2) thermal decomposition in organic medium (○, 30 mT, 700 kHz; •, 26 mT, 700 kHz) [Citation41], 3) thermal decomposition in organic medium followed by seed growth (+, 26 mT–700 kHz) [Citation37]. (B) Evolution of SAR (f = 54 kHz) as a function of magnetic field amplitude for two samples of different sizes (data extracted from Mehdaoui et al. [Citation49]). (C) Illustration of three different samples (S1, S2 and S3) with different sizes and size distributions and how the optimum frequency (f1, f2 and f3) for the maximum SAR is affected.
![Figure 3. (A) Comparison between the experimental SAR values (W/g Fe) of nanoparticles of different sizes obtained by three different synthetic approaches: 1) co-precipitation in water followed by a size selection process (□, 31 mT, 700 kHz) [Citation42], 2) thermal decomposition in organic medium (○, 30 mT, 700 kHz; •, 26 mT, 700 kHz) [Citation41], 3) thermal decomposition in organic medium followed by seed growth (+, 26 mT–700 kHz) [Citation37]. (B) Evolution of SAR (f = 54 kHz) as a function of magnetic field amplitude for two samples of different sizes (data extracted from Mehdaoui et al. [Citation49]). (C) Illustration of three different samples (S1, S2 and S3) with different sizes and size distributions and how the optimum frequency (f1, f2 and f3) for the maximum SAR is affected.](/cms/asset/adf9e3b1-cb7c-4372-8a12-2eda6c0c99e4/ihyt_a_826824_f0003_b.jpg)
To tackle the final goal of using MNPs in humans it would be highly desirable to develop methods for mass production for the industrial-scale manufacture of large quantities of product. The co-precipitation route has been already scaled up following the Massart procedure or its modifications [Citation43,Citation44]. The Sugimoto procedure seems to be very promising for obtaining larger particles because of the use of water and cheap reactants [Citation32], and work concerning the scaling up of this process is in progress in our group. More challenging is the scaling-up of the thermal decomposition process, for which some methodologies in the multi-gram scale have been described but much effort is still needed to preserve high-quality properties and reproducibility [Citation38,Citation45].
Particle size, size distribution and shape
Size
It is well established that the size of MNPs has a big impact on the heating efficiency since the power dissipation, P, of a given sample is related to the relaxation time:
where χ0 = initial DC susceptibility, H0 = amplitude of the applied field, µ0 = permeability of free space, f = frequency of the applied field and τ = relaxation time. Relaxation time is related to the size of nanoparticles, among other parameters, as is shown in Equations 3 and 4 (where τB = Brownian relaxation, τN = Néel relaxation, η = viscosity, VH =hydrodynamic volume, V = magnetic volume, kB = Boltzmann constant, T = temperature, and K = magnetic anisotropy energy density). Néel relaxation (Equation 3) time (τN) increases with magnetic volume, V, while Brownian relaxation (Equation 2) time (τB) is proportional to the hydrodynamic volume, VH, which is the size of the particles in suspension [Citation46]. The former is directly related to the MNPs core size while the latter is related to the size of the core, the aggregate and the coating. Relaxation depends on the volume of the particles, so small changes in diameter make huge differences in SAR. Thus, a change in diameter from 13 to 15 nm (15%) leads to a 50% increase in volume for cubic shaped particles and 35 % for spherical. Concerning the other mechanisms of heat generation commented in the introduction, AC hysteresis losses can be important if nanoparticles are ferromagnetic, while eddy currents are expected to be low for particles with diameters smaller than 200 nm [Citation47].
It should be highlighted that, given the amplitude (H) and frequency (f) of an applied alternating magnetic field, the dependence of SAR with size is not necessarily linear for measurements performed over a wide enough size range [Citation9,Citation40,Citation41,Citation48,Citation49]. Indeed, experimental data in the literature show that, for any given frequency, SAR increases with size to reach a maximum, after which larger particles exhibit less heat dissipation efficiency (). The particle size at which maximum SAR is attained increases with the amplitude of the magnetic field [Citation49].
Size distribution
Closely related with the issue of size is that of size distribution, which must be considered in order to explain hyperthermia results [Citation50,Citation51]. Its influence on the calorific power is two-fold: 1) samples with broad size distributions have many particles with different sizes and, consequently potentially different efficiencies for heat dissipation as discussed above, and 2) MNPs with non-similar sizes may have distinct saturation magnetisation values and anisotropies, also affecting the calorific power. So, a polydispersed sample will have in general a reduced maximum SAR value but may produce calorific output over a broader frequency range. However, monodispersed samples will have enhanced SAR values but only at the right frequency (). This has been calculated theoretically [Citation42,Citation46,Citation52] and quantified experimentally [Citation39]. For particles of similar average size, the SAR value significantly increases, as does the MS value (from 52 emu/g to 69 emu/g) when the size distribution becomes narrower (from 14 ± 1 nm in diameter to 15 ± 4 nm) ().
Figure 4. Difference in SAR values (50 mT, 77 kHz) between two samples synthesised by thermal decomposition in 1-octadecene, with the same mean size but different size distribution. Scale bars = 50 nm.
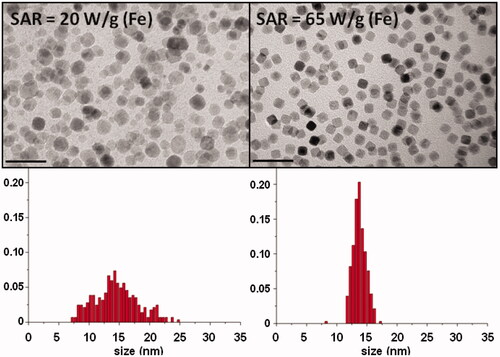
For hyperthermia studies it would be highly desirable to use the narrowest possible size distributions for a better understanding of the phenomena and a more homogenous heating effect. In other words, for this application the synthesis of MNPs by thermal decomposition seems to be ideal. This method allows the obtaining of particles with standard deviations in size lower than 10%, while size selection is needed if the co-precipitation route is chosen.
Shape
MNP shape affects magnetic and magneto-thermal properties through its influence on the saturation magnetisation and magnetic anisotropy. Spherical particles have a higher number of defects at the surface than cubic particles, which affect both properties: saturation magnetisation and magnetic anisotropy. The saturation magnetisation of Zn0.4Fe2.6O4 MNPs was found to be higher for nanocubes than for nanospheres of the same volume (18 and 22 nm of edge and diameter, respectively) [Citation53]. Analogous results were reported by Gonzalez-Fernandez et al. for cubic and spherical magnetite MNPs of similar size around 45 nm () [Citation33] and for nanoparticles synthesised by thermal decomposition in organic media [Citation54].
Figure 5. Difference in SAR values between two samples with similar mean size but different shape [Citation33].
![Figure 5. Difference in SAR values between two samples with similar mean size but different shape [Citation33].](/cms/asset/86c507de-d5f6-4d97-9e61-bdc1746e4057/ihyt_a_826824_f0005_b.jpg)
Maghemite flower-like MNPs have been synthesised by following a polyol method and their heating efficiency measured [Citation55]. These nanoflowers were composed of smaller grains of 11 nm in intimate contact with very small misalignments between the grains. Indeed most of the nanoflowers appear as single crystalline by HRTEM. In general, their heating efficiency (H = 27 mT, f = 700 kHz) increases with size (in the range 22–55 nm) and is always greater than the individual 11 nm crystallites obtained by a similar polyol method. Nanoflowers synthesised with long reaction times present better alignment of the individual crystallites providing the highest SAR values. Those values are closed to the ones reported for MNPs of 16.5 nm synthesised by co-precipitation and subjected to a size selection process (H = 31 mT, f = 700 kHz) [Citation42].
Also, the heating efficiency of those nanoflowers can be compared with nanocubes of similar sizes synthesised by thermal decomposition in organic medium, and examined under the same conditions of amplitude and frequency of the applied alternating field () [Citation41]. Thus, with H = 27–30 mT and f = 700 kHz, nanoflowers of 38 nm exhibit a heating efficiency considerably greater than nanocubes of the same size. It seems clear that larger fields are required to maximise the loss in those cubes. However, nanocubes of 19 nm are more efficient than any other studied nanocube or nanoflower.
Figure 6. SAR values for nanoflowers (solid, hollow and crossed circles correspond to particles synthesised at different experimental conditions) and nanocubes (solid squares), under similar field conditions. TEM micrographs adapted with permission from Guardia et al. [Citation41] and Hugounenq et al. [Citation55].
![Figure 6. SAR values for nanoflowers (solid, hollow and crossed circles correspond to particles synthesised at different experimental conditions) and nanocubes (solid squares), under similar field conditions. TEM micrographs adapted with permission from Guardia et al. [Citation41] and Hugounenq et al. [Citation55].](/cms/asset/c68557c8-8488-409f-932c-9346f289695d/ihyt_a_826824_f0006_b.jpg)
Chemical composition
Iron oxide MNPs are most often composed of variable proportions of maghemite (γ-Fe2O3) and magnetite (Fe3O4). Both have magnetic properties making them suitable for biomedical applications but magnetite has higher values of MS and anisotropy [Citation56], so the proportion between both has an impact on the magnetic properties. On the other hand the normal chemical evolution of Fe3O4 into the body is to be slowly oxidised to Fe2O3. For this reason some reports propose a pre-oxidation of the material before using it in biological samples.
The γ-Fe2O3/Fe3O4 proportion has been shown to depend on slight changes of the experimental parameters in MNPs synthesised by co-precipitation [Citation57] and by thermal decomposition [Citation38]. In addition, the formation of an FeO phase in the thermal decomposition of Fe(oleate)3 has been recently reported [Citation58], so the experimental parameters must be carefully chosen to avoid this undesired result.
Ferrites of the type MFe2O4 have also been employed for hyperthermia research. Changes in ferrite composition can change loss mechanism. For example, cobalt ions can increase saturation magnetisation and anisotropy, increasing the heating potential of ferrites [Citation59,Citation60]. Indeed, CoFe2O4 MNPs obtained by co-precipitation and stabilised with citrate exhibited superior heating properties than their analogous counterparts of maghemite [Citation42]. However, there are concerns about the use of such CoFe2O4 MNPs because of their potential toxicity. In spite of these concerns, Cheon et al. synthesised a set of nanoparticles of the types MFe2O4 and core@shell MFe2O4@MFe2O4 (M = Mn, Fe, Co) (size = 15 nm) and found SAR values (47 mT, 500 kHz) as high as 3,000 W/g with the following trend: CoFe2O4@Fe3O4 < CoFe2O4@MnFe2O4 < Fe3O4@CoFe2O4 < MnFe2O4@CoFe2O4 < Zn0.4Co0.6Fe2O4@Zn0.4Mn0.6Fe2O4. The in vitro cytotoxicity, after 24 h, of CoFe2O4@MnFe2O4 and MnFe2O4@CoFe2O4 core-shell MNPs was studied finding no problems of cell viability. CoFe2O4@MnFe2O4 were then tested for in vivo hyperthermia experiments with mice obtaining promising results [Citation61], and the magnetothermal response of Zn0.4Fe2.6O4-based nanomaterials was recently used for drug delivery in mice [Citation15].
Colloidal properties, magnetic interaction and media viscosity
The final goal in magnetic hyperthermia research is to use nanoparticles in the form of colloidal suspension injected into human beings. Therefore the challenge is not only to develop magnetic nanoparticles with good heating capacities, but also with good colloidal properties, long blood circulation times and with grafted ligands able to facilitate their specific internalisation in tumour cells.
Surface coating is the way for introducing functional groups to anchor biomolecules to the nanoparticle surface. Coating may affect the nanoparticle magnetic properties due to a partial dissolution of the particle during the process (which will lead to a reduction in MS) [Citation62]. However, when particle size is preserved, coating with carboxylic acids leads to the formation of strong bonds at the nanoparticle surface, reducing spin canting and increasing saturation magnetisation [Citation63]. But coating also determines to a great extent the biodistribution. Magnetic hyperthermia requires an accumulation of the nanoparticles at the tumour area that have not been achieved up to now by intravenous injection in comparison to intratumoral injection. Biodistribution may vary the aggregation state of the MNPs and therefore the magnetic interactions between them, and the viscosity media will depend on the organ or tissue of accumulation.
Interactions between magnetic nanoparticles involve dipole–dipole interactions and can have an important influence on their hyperthermia performance. They are affected by concentration and have a great influence on the magnetic relaxation and the susceptibility [Citation64]. It has been shown that there exists an optimal concentration for which the SAR is maximum [Citation65]. Dipole–dipole interactions can occur between 1) strongly bounded particles (i.e. throughout aggregates of particles), or 2) between aggregates and/or individual particles. In the latter case, interactions depend on concentration when dilution leads to separated particles reducing the agglomerate size. However, when dilution separates aggregates, interactions within the aggregates rule the magnetic behaviour (). On the other hand, size, shape and proximity between particles within the aggregate are also crucial factors to take into account for understanding the heating efficiency of a colloidal suspension, as it was shown for nanoflowers [Citation55].
It has been proposed that these interactions may enhance the heating efficiency by dipolar coupling under an oscillating magnetic field and by increasing local temperature due to the increase in the local concentration and anisotropy [Citation66,Citation67]. Larger particles have larger interactions and agglomeration is more likely at higher concentrations. However, these interactions do not always favour heat generation. For 30 nm nanoparticles, concentration plays a relevant role in the calorific output due to the larger interactions. As concentration increases, SAR first increases up to a maximum and then decreases [Citation68]. With smaller nanoparticles between 6 and 14 nm, no variation of SAR was found in a broad range of concentrations () [Citation69].
Figure 8. SAR (W/g Fe) versus concentration of MNPs of various sizes (concentration scale is logarithmic). Full circles: 30 nm (249 kHz, 12.5 mT) [Citation68]. Empty circles, squares and triangles: 13, 11 and 8 nm, respectively (522.7 kHz, 9.4 mT) [Citation69].
![Figure 8. SAR (W/g Fe) versus concentration of MNPs of various sizes (concentration scale is logarithmic). Full circles: 30 nm (249 kHz, 12.5 mT) [Citation68]. Empty circles, squares and triangles: 13, 11 and 8 nm, respectively (522.7 kHz, 9.4 mT) [Citation69].](/cms/asset/5e37315c-0b5f-41cf-8eeb-36f96738377b/ihyt_a_826824_f0008_b.jpg)
This size-dependent influence of concentration on SAR seems to be widespread, as recently reported for big particles [Citation21,Citation54]. In a similar way to the behaviour reported above, SAR values exhibit a maximum for particles of 37 and 65 nm (shifted to lower concentration for the latter) while it just decreases with concentration when the particles are 150 nm in size.
The influence of concentration lies in dipole–dipole interactions, whether they are beneficial or detrimental for hyperthermia, that increase with size and have been confirmed by susceptibility measurements, as well as have been confirmed the relationship between them, magnetic remanence and hysteresis losses [Citation22]. Thus, the nanoflowers discussed before can be seen as irreversible aggregates of smaller individual particles of 11 nm in very intimate contact. From this point of view that kind of aggregation leads to a huge increase in SAR [Citation23].
Finally, viscosity directly affects the Brownian relaxation and some authors have evaluated SAR values in different solvents and mixtures, or even in agar media to simulate the viscosity of the cell. In all cases the general trend is a progressive reduction of SAR values when increasing viscosity, as observed for MNPs in aqueous and agar suspensions [Citation69] or in different glycols [Citation42,Citation70].
Conclusions and future directions
Recent developments indicate that magnetic hyperthermia could help to improve clinical practice in the treatment of cancer, most probably in synergy with other conventional treatments. Significant advances in the field of magnetic hyperthermia mediators have been presented here and the complexity of the problem has been highlighted. There already exist methods to obtain magnetic nanoparticles with the appropriate properties to be used as hyperthermia agents, bearing in mind that these properties must be optimised to suit the magnetic field and application frequency that will be used. The lack of conclusive data comparing the predicted and the in vivo performance makes it difficult to decide an optimum MNP size, which will also depend on the experimental conditions of the magnetic field.
The scaling-up of the synthesis by thermal decomposition in organic media remains a challenge. More promising seems to be the mass production of larger particle sizes by the controlled oxidation of an Fe(II) salt in water.
Additionally, the coating and the colloidal properties must be carefully taken into account, which can be a difficult task for some of the large magnetic particles. Nanoparticle functionalisation to specifically target the hyperthermia agent to a tumour ensuring complete removal of the malignant cells while preserving the healthy ones remains a challenge. Up to now, accumulation of the nanoparticles at the tumour area has been achieved by intratumoral injection.
Declaration of interest
This work was partially supported by projects from the Spanish Ministry of Economy and Competitiveness (MAT2011-23641 and CSD2007-00010 to MPM), the Madrid regional government CM (S009/MAT-1726 to MPM) and EU-FP7 MULTIFUN project (NMP-Large ref. 246479). The authors alone are responsible for the content and writing of the paper.
References
- Arias JL, Reddy LH, Othman M, Gillet B, Desmaële D, Zouhiri F, et al. Squalene based nanocomposites: A new platform for the design of multifunctional pharmaceutical theragnostics. ACS Nano 2011;5:1513–21
- Kohler N, Sun C, Fichtenholtz A, Gunn J, Fang C, Zhang M. Methotrexate-immobilized poly(ethylene glycol) magnetic nanoparticles for MR imaging and drug delivery. Small 2006;2:785–92
- Colombo M, Carregal-Romero S, Casula MF, Gutiérrez L, Morales MP, Böhm IB, et al. Biological applications of magnetic nanoparticles. Chem Soc Rev 2012;41:4306–34
- Jordan A, Scholz R, Wust P, Fa H, Felix R. Magnetic fluid hyperthermia (MFH): Cancer treatment with AC magnetic field induced excitation of biocompatible superparamagnetic nanoparticles. J Magn Magn Mater 1999;201:413–19
- Hilger I, Hergt R, Kaiser WA. Towards breast cancer treatment by magnetic heating. J Magn Magn Mater 2005;293:314–19
- Ivkov R, DeNardo SJ, Daum W, Foreman AR, Goldstein RC, Nemkov VS, et al. Application of high amplitude alternating magnetic fields for heat induction of nanoparticles localized in cancer. Clinical Cancer Research 2005;11:S7093–103
- Maier-Hauff K, Ulrich F, Nestler D, Niehoff H, Wust P, Thiesen B, et al. Efficacy and safety of intratumoral thermotherapy using magnetic iron-oxide nanoparticles combined with external beam radiotherapy on patients with recurrent glioblastoma multiforme. J Neurooncol 2011;103:317–24
- Thiesen B, Jordan A. Clinical applications of magnetic nanoparticles for hyperthermia. Int J Hyperthermia 2008;24:467–74
- Krishnan KM. Biomedical nanomagnetics: A spin through possibilities in imaging, diagnostics, and therapy. IEEE Trans Magn 2010;46:2523–58
- Ortega D, Pankhurst QA. Magnetic hyperthermia. In: O’Brien P, ed. Nanoscience: Vol 1: Nanostructures through Chemistry. Cambridge: Royal Society of Chemistry, 2013, pp. 60–88
- Hilger I, Kaiser WA. Iron oxide-based nanostructures for MRI and magnetic hyperthermia. Nanomedicine 2012;7:1443–59
- Golneshan AA, Lahonian M. The effect of magnetic nanoparticle dispersion on temperature distribution in a spherical tissue in magnetic fluid hyperthermia using the lattice Boltzmann method. Int J Hyperther 2011;27:266–74
- Attaluri A, Ma R, Qiu Y, Li W, Zhu L. Nanoparticle distribution and temperature elevations in prostatic tumours in mice during magnetic nanoparticle hyperthermia. Int J Hyperthermia 2011;27:491–502
- Owen J, Pankhurst Q, Stride E. Magnetic targeting and ultrasound mediated drug delivery: Benefits, limitations and combination. Int J Hyperthermia 2012;28:362–73
- Wang H, Li X, Xi X, Hu B, Zhao L, Liao Y, et al. Effects of magnetic induction hyperthermia and radiotherapy alone or combined on a murine 4T1 metastatic breast cancer model. Int J Hyperthermia 2011;27:563–72
- Lee J-H, Chen K-J, Noh S-H, Garcia MA, Wang H, Lin W-Y, et al. On-demand drug release system for in vivo cancer treatment through self-assembled magnetic nanoparticles. Ang Chem Int Ed 2013;52:4384–88
- Reddy LH, Arias JL, Nicolas J, Couvreur P. Magnetic nanoparticles: Design and characterization, toxicity and biocompatibility, pharmaceutical and biomedical applications. Chem Rev 2012;112:5818–78
- Hergt R, Dutz S, Zeisberger M. Validity limits of the Néel relaxation model of magnetic nanoparticles for hyperthermia. Nanotechnology 2010;21:015706
- Neuweltb EA, Papisov M, Weissleder R, Bogdanov A. Long-circulating iron oxides for MR imaging. Adv Drug Deliv Rev 1995;16:321–4
- Bigall NC, Wilhelm C, Beoutis M-L, Garcia-Hernandez M, Khan AA, Giannini C, et al. Colloidal ordered assemblies in a polymer shell – A novel type of magnetic nanobeads for theranostic applications. Chem Mater 2013;25:1055–62
- Martinez-Boubeta C, Simeonidis K, Serantes D, Conde-Leborán I, Kazakis I, Stefanou G, et al. Adjustable hyperthermia response of self-assembled ferromagnetic FeMgO core-shell nanoparticles by tuning dipole–dipole interactions. Adv Funct Mater 2012;22:3737–44
- Serantes D, Baldomir D, Martinez-Boubeta C, Simeonidis K, Angelakeris M, Natividad E, et al. Influence of dipolar interactions on hyperthermia properties of ferromagnetic particles. J Appl Phys 2010;108:073918
- Lartigue L, Hugounenq P, Alloyeau D, Clarke SP, Lévy M, Bacri JC, et al. Cooperative organization in iron oxide multi-core nanoparticles potentiates their efficiency as heating mediators and MRI contrast agents. ACS Nano 2012;6:10935–49
- Tartaj P, Morales MP, Gonzalez-Carreño T, Veintemillas-Verdaguer S, Serna CJ. The iron oxides strike back: From biomedical applications to energy storage devices and photoelectrochemical water splitting. Adv Mater 2011;23:5243–9
- Pankhurst QA, Thanh NKT, Jones SK, Dobson J. Progress in applications of magnetic nanoparticles in biomedicine. J Phys D: Appl Phys 2009;42:224001
- Maity D, Kale SN, Kaul-Ghanekar R, Xue J-M, Ding J. Studies of magnetite nanoparticles synthesized by thermal decomposition of iron (III) acetylacetonate in tri(ethylene glycol). J Magn Magn Mater 2009;321:3093–8
- Cabrera L, Gutierrez S, Menendez N, Morales MP, Herrasti P. Magnetite nanoparticles: Electrochemical synthesis and characterization. Electrochim Acta 2008;53:3436–1
- Verma A, Stellacci F. Effect of surface properties on nanoparticle-cell interactions. Small 2010;6:12–21
- Kettering M, Winter J, Zeisberger M, Bremer-Streck S, Oehring H, Bergemann C, et al. Magnetic nanoparticles as bimodal tools in magnetically induced labelling and magnetic heating of tumour cells: An in vitro study. Nanotechnology 2007;18:175101
- Huang H, Delikanli S, Zeng H, Ferkey DM, Pralle A. Remote control of ion channels and neurons through magnetic-field heating of nanoparticles. Nature Nanotech 2010;5:602–6
- Mornet S, Vasseur S, Grasset F, Duguet E. Magnetic nanoparticle design for medical diagnosis and therapy. J Mater Chem 2004;14:2161–75
- Sugimoto T, Matijevic E. Formation of uniform spherical magnetite particles by crystallization from ferrous hydroxide gels. J Coll Int Sci 1980;74:227–43
- Gonzalez-Fernandez MA, Torres TE, Andrés-Vergés M, Costo R, de la Presa P, Serna CJ, et al. Magnetic nanoparticles for power absorption: Optimizing size, shape and magnetic properties. J Solid State Chem 2009;182:2779–84
- Ma M, Wu Y, Zhou J, Sun Y, Zhang Y, Gu N. Size dependence of specific power absorption of Fe3O4 particles in AC magnetic field. J Magn Magn Mater 2004;268:33–9
- Jun Y, Choi J, Cheon J. Shape control of semiconductor and metal oxide nanocrystals through nonhydrolytic colloidal routes. Angew Chem Int Ed Eng 2006;45:3414–39
- Salas G, Costo R, Morales MP. Synthesis of inorganic nanoparticles. In: de la Fuente JM, Grazu V, eds. Frontiers in Nanoscience, Vol 4. Nanobiotechnology: Inorganic Nanoparticles vs Organic Nanoparticles. Amsterdam: Elsevier, 2012, pp. 35–79
- Levy M, Quarta A, Espinosa A, Figuerola A, Wilhelm C, García-Hernández M, et al. Correlating magneto-structural properties to hyperthermia performance of highly monodisperse iron oxide nanoparticles prepared by a seeded-growth route. Chem Mater 2011;23:4170–80
- Park J, An K, Hwang Y, Park J-G, Noh H-J, Kim J-Y, et al. Ultra-large-scale syntheses of monodisperse nanocrystals. Nature Mater 2004;3:891–5
- Salas G, Casado C, Teran FJ, Miranda R, Serna CJ, Morales MP. Controlled synthesis of uniform magnetite nanocrystals with high-quality properties for biomedical applications. J Mater Chem 2012;22:21065–75
- Guardia P, Pérez-Juste J, Labarta A, Batlle X, Liz-Marzán LM. Heating rate influence on the synthesis of iron oxide nanoparticles: The case of decanoic acid. Chem Commun 2010;46:6108–10
- Guardia P, Di Corato R, Lartigue L, Wilhelm C, Espinosa A, Garcia-Hernandez M, et al. Water-soluble iron oxide nanocubes with high values of specific absorption rate for cancer cell hyperthermia treatment. ACS Nano 2012;6:3080–91
- Fortin JP, Wilhelm C, Servais J, Ménager C, Bacri JC, Gazeau F. Size-sorted anionic iron oxide nanomagnets as colloidal mediators for magnetic hyperthermia. J Am Chem Soc 2007;129:2628–35
- Massart R. Preparation of aqueous magnetic liquids in alkaline and acidic media. IEEE Trans Magn 1981;17:1247–8
- Molday RS. Magnetic iron-dextran microspheres. Patent US4452773, 1984
- Hyeon T, Park J, Piao Y. Method of preparing iron oxide nanoparticles coated with hydrophilic material, and magnetic resonance imaging contrast agent using the same. WO2012108648, 2012 (Hanwha Chemical Corporation, Seoul, South Korea)
- Gonzales-Weimuller M, Zeisberger M, Krishnan KM. Size-dependant heating rates of iron oxide nanoparticles for magnetic fluid hyperthermia. J Magn Magn Mater 2009;321:1947–50
- Ramprasad R, Zurcher P, Petras M, Miller M, Renaud P. Magnetic properties of metallic ferromagnetic nanoparticle composites. J Appl Phys 2004;96:519–29
- Khandhar AP, Ferguson RM, Krishnan KM. Monodispersed magnetite nanoparticles optimized for magnetic fluid hyperthermia: Implications in biological systems. J App Phys 2011;109:07B310
- Mehdaoui B, Meffre A, Carrey J, Lachaize S, Lacroix L-M, Gougeon M, et al. Optimal size of nanoparticles for magnetic hyperthermia: A combined theoretical and experimental study. Adv Funct Mater 2011;21:4573–81
- Rosensweig RE. Heating magnetic fluid with alternating magnetic field. J Magn Magn Mater 2002;252:370–74
- Lartigue L, Innocenti C, Kalaivani T, Awwad A, Sanchez Duque MDM, Guari Y, et al. Water-dispersible sugar-coated iron oxide nanoparticles. An evaluation of their relaxometric and magnetic hyperthermia properties. J Am Chem Soc 2011;133:10459–72
- Hergt R, Dutz S, Röder M. Effects of size distribution on hysteresis losses of magnetic nanoparticles for hyperthermia. J Phys: Condens Matter 2008;20:385214
- Noh S-H, Na W, Jang J-T, Lee J-H, Lee EJ, Moon SH, et al. Nanoscale magnetism control via surface and exchange anisotropy for optimized ferrimagnetic hysteresis. Nano Lett 2012;12:3716–21
- Martinez-Boubeta C, Simeonidis K, Makridis A, Angelakeris M, Iglesias O, Guardia P, et al. Learning from nature to improve the heat generation of iron-oxide nanoparticles for magnetic hyperthermia applications. Sci Rep 2013;3:1652
- Hugounenq P, Levy M, Alloyeau D, Lartigue L, Dubois E, Cabuil V, et al. Iron oxide monocrystalline nanoflowers for highly efficient magnetic hyperthermia. J Phys Chem C 2012;116:15702–12
- Cornell RM, Schwertmann U. The Iron Oxides. Structure, Properties, Reactions, Occurrences and Uses. 2nd Ed. Weinheim, Germany: Wiley-VCH, 2003, p. 123
- Santoyo Salazar J, Perez L, de Abril O, Truong Phuoc L, Ihiawakrim D, Vazquez M, et al. Magnetic iron oxide nanoparticles in 10−40 nm range: Composition in terms of magnetite/maghemite ratio and effect on the magnetic properties. Chem Mater 2011;23:1379–86
- Pichon BP, Gerber O, Lefevre C, Florea I, Fleutot S, Baaziz W, et al. Microstructural and magnetic investigations of wüstite-spinel core-shell cubic-shaped nanoparticles. Chem Mater 2011;23:2886–900
- Veverka M, Veverka P, Kaman O, Lančok A, Závěta K, Pollert E, et al. Magnetic heating by cobalt ferrite nanoparticles. Nanotechnology 2007;18:345704
- Verde EL, Landi GT, Gomes JA, Sousa MH, Bakuzis AF. Magnetic hyperthermia investigation of cobalt ferrite nanoparticles: Comparison between experiment, linear response theory, and dynamic hysteresis simulations. J Appl Phys 2012;111:123902
- Lee J-H, Jang J, Choi J, Moon SH, Noh S, Kim J, et al. Exchange-coupled magnetic nanoparticles for efficient heat induction. Nat Nano 2011;6:418–22
- de Sousa ME, Fernández van Raap MB, Rivas PC, Mendoza Zélis P, Girardin P, Pasquevich GA, et al. Stability and relaxation mechanisms of citric acid coated magnetite nanoparticles for magnetic hyperthermia. J Phys Chem C 2013;117:5436–45
- Salafranca J, Gazquez J, Pérez N, Labarta A, Pantelides ST, Pennycook SJ, et al. Surfactant organic molecules restore magnetism in metal-oxide nanoparticle surfaces. Nano Lett 2012;12:2499–503
- Majetich SA, Sachan M. Magnetostatic interactions in magnetic nanoparticle assemblies: Energy, time and length scales. J Phys D: Appl Phys 2006;39:R407–22
- Haase C, Nowak U. Role of dipole–dipole interactions for hyperthermia heating of magnetic nanoparticle ensembles. Phys Rev B 2012;85:045435
- Dennis CL, Jackson AJ, Borchers JA, Hoopes PJ, Strawbridge R, Foreman AR, et al. Nearly complete regression of tumors via collective behavior of magnetic nanoparticles in hyperthermia. Nanotechnology 2009;20:395103
- Mehdaoui B, Tan RP, Meffre A, Carrey J, Lachaize S, Chaudret B, et al. Increase of magnetic hyperthermia efficiency due to dipolar interactions in low-anisotropy magnetic nanoparticles: Theoretical and experimental results. Phy Rev B 2013;87:174419
- Andrés Vergés M, Costo R, Roca AG, Marco JF, Goya GF, Serna CJ, et al. Uniform and water stable magnetite nanoparticles with diameters around the monodomain–multidomain limit. J Phys D: Appl Phys 2008;41:134003
- de la Presa P, Luengo Y, Multigner M, Costo R, Morales MP, Rivero G, et al. Study of heating efficiency as a function of concentration, size, and applied field in γ-Fe2O3 nanoparticles. J Phys Chem C. 2012;116:25602–10
- Piñeiro-Redondo Y, Bañobre-López M, Pardiñas-Blanco I, Goya G, López-Quintela MA, Rivas J. The influence of colloidal parameters on the specific power absorption of PAA-coated magnetite nanoparticles. Nanoscale Res Lett 2011;6:383