Abstract
Ultrasound contrast agents (UCAs) are used routinely in the clinic to enhance contrast in ultrasonography. More recently, UCAs have been functionalised by conjugating ligands to their surface to target specific biomarkers of a disease or a disease process. These targeted UCAs (tUCAs) are used for a wide range of pre-clinical applications including diagnosis, monitoring of drug treatment, and therapy. In this review, recent achievements with tUCAs in the field of molecular imaging, evaluation of therapy, drug delivery, and therapeutic applications are discussed. We present the different coating materials and aspects that have to be considered when manufacturing tUCAs. Next to tUCA design and the choice of ligands for specific biomarkers, additional techniques are discussed that are applied to improve binding of the tUCAs to their target and to quantify the strength of this bond. As imaging techniques rely on the specific behaviour of tUCAs in an ultrasound field, it is crucial to understand the characteristics of both free and adhered tUCAs. To image and quantify the adhered tUCAs, the state-of-the-art techniques used for ultrasound molecular imaging and quantification are presented. This review concludes with the potential of tUCAs for drug delivery and therapeutic applications.
Introduction
Ultrasound contrast agents (UCAs) consist of gas bubbles that are typically stabilised by an albumin, lipid or polymer shell. For over three decades, UCAs have been clinically used to enhance ultrasound (US) imaging in different fields, such as cardiology and radiology [Citation1,Citation2]. Targeted UCAs (tUCAs) differ from clinically approved UCAs by the decoration of their shell with targeting ligands [Citation3]. Due to their typical size (∼1–10 µm) UCAs are confined to the blood pool only [Citation4,Citation5]. This makes tUCAs ideal agents to adhere to intravascular biomarkers expressed on endothelial cells, to target, for instance, cancer and cardiovascular disease, as covered in this review. Because of the large compressibility of the gas core of the microbubbles (MBs), they create non-linear backscatter and reflection in an US field, allowing for differentiation between the highly echogenic agent and surrounding tissues and fluids [Citation6,Citation7]. Recently, smaller tUCAs have been synthesised which allow them to extravasate out of leaky vasculature offering opportunities for new applications [Citation8,Citation9].
This review focuses on tUCAs for ultrasound molecular imaging (UMI) and therapy. Manufacturing and functionalising tUCAs will be covered, including targeting novel biomarkers. The binding and acoustic properties of bound tUCAs will be evaluated, as these properties are important for both UMI and therapy. The current state-of-the-art clinical and preclinical molecular imaging techniques and quantification methods are discussed. tUCA-mediated drug and gene delivery is a relatively new field as the first proof of concept was reported in 2011 [Citation10]. The progress since then will be presented.
Targeting and binding of tUCAs
To compose tUCAs several aspects have to be accounted for. A choice for the coating has to be made, the biomarkers that are upregulated in the disease of interest have to be identified, and a suitable targeting ligand has to be found. This targeting ligand has to be attached to the contrast agent and the binding properties of the tUCA have to be evaluated.
Coating materials
The commercially available UCAs have different coating materials to reduce the surface tension and gas diffusion out of the UCAs, thereby increasing their lifetime. These are: albumin (Albunex, Cardiosphere, Optison), galactose (Echovist, Levovist), lipids (Definity, Imagent, Levovist, Lumason, MicroMarker, Sonazoid, SonoVue, Targestar), or polymers (Acusphere, Sonovist) [Citation11,Citation12]. The main advantage of lipid-coated bubbles is that different mixtures can be easily formulated and modified [Citation12]. Very recently, super-resolution fluorescence microscopy revealed that the main lipid in the coating (1,2-distearoyl-sn-glycero-3-phosphocholine (DSPC) or 1,2-dipalmitoyl-sn-glycero-3-phosphocholine (DPPC)) influences the ligand distribution on the shell [Citation13], and it was also shown that the difference in ligand distribution also changes the acoustical properties [Citation14]. This offers opportunities for designing UCAs with very specific and known properties. Most lipid-coated UCAs have a brush of polyethylene glycol (PEG) incorporated into their shell to prevent close contact between neighbouring bubbles to inhibit their fusion and to shield them from the immune system [Citation12]. But although this is generally accepted as a method to increase UCA lifetime, the necessity of incorporating PEG for tUCAs has been questioned [Citation15]. This study indicated that small peptides, either conjugated to the lipid directly or via a PEG-spacer (∼10 monomers), might hinder access of the ligand to the target receptors when short PEG brushes (molecular weight of 350 kDa; i.e. 8 monomers) are part of the coating. Even when the MBs were prepared without a PEG brush in their coating, the introduction of a PEG spacer between the ligand and the lipid seemed to reduce binding. Their hypothesis is that the flexibility of the spacer possibly enabled the peptide to loop back onto the bubble surface. According to Marsh et al. [Citation16] this is indeed what happens; due to their choice of incorporating relatively short PEG chains in the coating, the PEG chains will be in the mushroom regime and therefore assume a random configuration. If they had used similar concentrations of PEG with longer chains, such as PEG(2000) containing 45 monomers, these chains would be in the brush state, a more stretched and less random configuration [Citation16]. The random configuration of the PEG chain in the study of Myrset [Citation15] could thus shield the ligand, whilst a brush configuration may have been advantageous.
Polymer tUCAs have a stiffer and thicker coating than lipid-coated bubbles, and the main acoustic difference is their echogenicity; usually a polymer bubble is destroyed and the free gas bubble is detected, whereas a lipid-coated bubble can be used for non-destructive imaging [Citation6]. Combinations of different shell materials have also been reported; Ottoboni et al. [Citation17] used microcapsules with a cross-linked albumin outer layer and a poly-(DL-lactide) inner layer. The advantage of the two different layers is the possibility to tweak the acoustic performance via the inner polymer layer in terms of their stiffness and thickness, and to change the biological activity via the protein outer layer.
In vivo, the adsorption of serum proteins on the shell (opsonisation) is a major challenge in the design of UCAs, as it might inhibit binding of the tUCa to its target. Lipid-coated MBs based on phosphatidylcholine (PC) – especially pure DSPC – have lower serum protein adsorption, higher stability in serum, and lower uptake by the reticuloendothelial system (RES) than negatively charged phospholipids, such as phosphatidylserine (PS) [Citation15]. Another problem caused by opsonisation is its triggering capacity for phagocytosis. As targeting ligands typically present nucleophilic groups (e.g. hydroxyl or amino), this could trigger complement C3/C3b activation to promote phagocytosis and decrease the tUCA circulation time. This can be partly overcome by using longer PEG chains that are in the brush state [Citation16] to shield the ligands from complement activation (‘overbrushing’) and thus reduce immunogenicity [Citation18]. Unnikrishnan and Klibanov [Citation12] on the other hand, state that complement activation aids MB adherence in the microvasculature. Although PEGylation of UCAs might decrease the circulation time, it does reduce immunogenicity and thus seems to be desirable.
Attaching ligands
The ligand that makes the tUCA functional is typically a peptide, protein, polymer, antibody, nanobody or aptamer [Citation11,Citation19–21] (). A reactive moiety suitable for conjugation with the ligand of interest needs to be attached to the tUCA shell, of which biotin (non-covalent), or covalent coupling via a carboxylate group, thiol, or maleimide are most common [Citation12,Citation22] (). Covalent coupling does not require foreign proteins such as streptavidin, so the chances of immune response in a clinical setting are low [Citation23]. A carboxyl group incorporated in the tUCA shell can be activated with carbodiimide, forming an active ester that reacts with the protein amino group, forming an amide bond. However, proteins possess multiple lysine residues, so coupling via amide bonds is random and may therefore interfere with ligand-receptor interaction. As an alternative, a maleimide on the shell is coupled to a thiol group on the ligand (or vice versa), forming a thioether. The advantage of maleimide-thiol coupling is oriented coupling: a ligand protein possessing a single thiol then has a single point attachment to the bubble shell. This retains the affinity of the ligand to its target [Citation12]. A new class of ligands are camelid-derived single-domain antibody-fragments (∼15 kDa), nanobodies, that do not induce an immune response in humans [Citation20].
Figure 1. Targeting and binding of tUCAs. (A) tUCAs targeted to their specific biomarker via an antibody (the antibody is used as an example and can be replaced by other ligands, as mentioned in the text). A1 shows both free and adhered tUCAs in a blood vessel (RBC = red blood cell). A2 shows a detailed representation of a ligand that is coupled to the lipid shell via biotin-streptavidin bridging, where the ligand adheres to the biomarker. The red arrows in A3 point to an example of a non-covalent linker (i.e. biotin-streptavidin bridging) and a covalent linker. (B) A lipid-coated MB with a shell based on DSPC (B1) or DPPC (B2), where the DSPC-based MB has a smaller binding area (indicated by white arrow) and a more spherical shape than the DPPC-based MB (reprinted (adapted) from Kooiman et al. [Citation13], with permission from John Wiley and Sons). (C) StemBells before the application of US (left panel) are pushed towards the vessel wall due to acoustic radiation force (right panel) (reprinted (adapted) from Kokhuis et al. [Citation62] with permission from John Wiley and Sons). (D) A MB attached to a glass bead via two micropipettes. The force needed to separate them is used to measure the binding force (reprinted (adapted) with permission from Kim et al. [Citation63]. ©2014 American Chemical Society).
![Figure 1. Targeting and binding of tUCAs. (A) tUCAs targeted to their specific biomarker via an antibody (the antibody is used as an example and can be replaced by other ligands, as mentioned in the text). A1 shows both free and adhered tUCAs in a blood vessel (RBC = red blood cell). A2 shows a detailed representation of a ligand that is coupled to the lipid shell via biotin-streptavidin bridging, where the ligand adheres to the biomarker. The red arrows in A3 point to an example of a non-covalent linker (i.e. biotin-streptavidin bridging) and a covalent linker. (B) A lipid-coated MB with a shell based on DSPC (B1) or DPPC (B2), where the DSPC-based MB has a smaller binding area (indicated by white arrow) and a more spherical shape than the DPPC-based MB (reprinted (adapted) from Kooiman et al. [Citation13], with permission from John Wiley and Sons). (C) StemBells before the application of US (left panel) are pushed towards the vessel wall due to acoustic radiation force (right panel) (reprinted (adapted) from Kokhuis et al. [Citation62] with permission from John Wiley and Sons). (D) A MB attached to a glass bead via two micropipettes. The force needed to separate them is used to measure the binding force (reprinted (adapted) with permission from Kim et al. [Citation63]. ©2014 American Chemical Society).](/cms/asset/50bf358f-8cb6-4a7d-ac03-18aafe2e114b/ihyt_a_997809_f0001_c.jpg)
Biomarkers and targeting strategies
tUCAs can be decorated with ligands against various diseases. As typical UCAs are confined to the vascular tree [Citation5], the most commonly targeted biological processes are angiogenesis, inflammation, and thrombosis. With the introduction of smaller tUCAs that can extravasate, apoptosis can also be targeted [Citation24].
Angiogenesis
Generally, tumours can be targeted by means of biomarkers for angiogenesis: αvβ3 integrin, vascular endothelial growth factor (VEGF), vascular endothelial growth factor receptor 2 (VEGFR2), endoglin (CD105) [Citation11,Citation19,Citation25,Citation26], or a combination thereof [Citation27]. Cyclic RGD is a clinically translatable ligand that was confirmed to adhere to endothelial cells expressing αvβ3. In addition, the bubbles conjugated to cRGD had sufficient residence time to attach to the integrin and were specific for αvβ3-expressing cells [Citation28]. Another strategy to target αvβ3 used the clinically approved contrast agent Sonazoid. The PS incorporated in the UCA coating was conjugated to lactaderin. This is analogous to the process of phagocytosis: apoptotic cells externalise PS allowing lactadherin to bind to PS to promote binding to the integrins on the surface of phagocytic cells [Citation29]. Since adherence of the MBs functionalised with lactadherin to human umbilical vein endothelial cells (HUVEC) under flow was higher than for bare MBs, this complex has potential to be translated to the clinic for targeting angiogenesis.
For VEGFR2 targeting the lipid-coated BR55 bubble (Bracco Diagnostics, Milan, Italy) is most promising for translation to the clinic, for which a heterodimer peptide is directly conjugated to the PEGylated lipid [Citation25]. Recently, a phase 0 clinical trial with this agent for prostate cancer was successfully conducted [Citation30].
Inflammation
Inflammation plays a role in several diseases, such as atherosclerosis and transient ischaemia [Citation31–34]. Specific inflammation markers that have been used for tUCAs are intracellular adhesion molecule 1 (ICAM-1), vascular cell adhesion molecule 1 (VCAM-1), E-selectin, and P-selectin [Citation17,Citation19,Citation35,Citation36]. MBs targeted to VCAM-1 can be used to discriminate the severity of inflammatory burden in mice with various degrees of atherosclerosis [Citation31]. This suggests that assessment of early inflammation in plaques is feasible. However, the same study showed that MB attachment to endothelial cells exposed to high wall shear stresses was very low (in vitro). This did improve with short interruptions of the high shear rate, as is the case with a pulsatile blood flow. Since the adhesion molecules ICAM-1 and VCAM-1 mediate the firmer adhesion of the leukocytes to the endothelium, and E-selectin and P-selectin promote the initial attachment and rolling of leukocytes [Citation19], targeting both selectin and adhesion molecules can potentially improve initial binding and increase the binding strength.
Thrombosis
Targeting of thrombi is mainly focused on the glycoprotein IIb-IIIa (GPIIb-IIIa or CD41/CD61) expressed by activated platelets in the thrombus [Citation8,Citation11,Citation19,Citation37]. This glycoprotein mediates platelet aggregation and is the most abundant receptor on the platelet surface [Citation37]. Using Targestar-SA (Targeson Inc., San Diego, CA) conjugated with anti-GPIIb/IIIa single-chain antibodies, these bubbles bound specifically to activated platelets in vitro. This may allow for real-time in vivo molecular imaging of acute arterial thrombosis and monitoring of pharmacological thrombolysis. Next to antibodies, it has been shown that cRGD can also be used to target GPIIb-IIIa. Although this ligand is generally known as a marker for angiogenesis, cRGD was shown to be specific for GPIIb-IIIa (also known as integrin αiibβ3) as well [Citation38]. cRGD was conjugated to the MBs via thiol-maleimide coupling and binding to GPIIb-IIIa was evaluated in vitro in the presence of plasma and under wall shear stresses up to 8 dynes/cm2 [Citation8] – a value representative for the human aorta averaged over the heart cycle [Citation39]. Several studies have demonstrated that cRGD exhibits an ∼30 × higher binding efficiency than linear RGD, and indeed significantly more cRGD bubbles adhered up to the highest shear rate in vitro and in the larger arteries of mice [Citation8].
Nakatsuka et al. [Citation21] recently introduced a new concept using MBs that are only acoustically active at thrombin levels associated with clot formation. The targeting moiety of these bubbles was a thrombin aptamer crosslinking strand (TACS). Crosslinking limits the non-linear signal generation of the MB due to the immobilisation of the lipids in the shell. Upon de-crosslinking, the polymer-DNA strands completely displace from the TACS, allowing the MB to oscillate freely, enhancing their non-linear response. The MBs consisted of DSPC, DPPA (1,2-dipalmitoyl-sn-glycero-3-phosphate), and 1,2-distearoyl-sn-glycero-3-phosphoethanolamine-poly(acrylic acid)-TACS (DSPE-PAA-TACS). When the bubbles are in contact with thrombin in the thrombus, this protein binds to the aptamer, which results in de-crosslinking. The in vitro onset of de-crosslinking was about 20 nM thrombin. Since in vivo clot formation starts with a concentration of ∼25 nM thrombin this offers opportunities to detect small clots at an early stage [Citation21]. This has been shown in vivo using similar MBs, but with different amounts of DSPE-PEG(5000) added to the mixture [Citation40]. Small amounts of PEG were found to improve stability, while higher concentrations did not contribute significantly to stability. Indeed, it was also shown that these aptamer MBs enhanced US signal in the vicinity of clots.
Novel targeting strategies
In the last few years molecular imaging using MBs has emerged, and multiple novel targets have been proposed and investigated. Prostate cancer is difficult to diagnose non-invasively, and common practice is routine clinical testing in the laboratory to determine the level of prostate-specific antigen in the blood. However, this test lacks sensitivity and specificity [Citation41]. The prostate-specific membrane antigen (PSMA) has higher expression levels in prostate cancer epithelial tissue than in normal prostate tissue and benign prostatic hyperplasia [Citation42], and is therefore very promising for UMI and staging of prostate cancer as shown in vitro [Citation43]. Next to prostate cancer, other types of cancers have been successfully targeted in vivo using tUCA: (1) tumour vessels of angiosarcoma that were targeted via secreted frizzled related protein 2 (SFRP2) using DSPC:PEG(2000)-PE bubbles [Citation44]; (2) ovarian cancer tumour vasculature that expresses CD276 with Target-Ready MicroMarker conjugated to anti-CD276 [Citation45]; and (3) the neovasculature of pancreatic ductal adenocarcinoma targeted to thymocyte differentiation antigen 1 (Thy-1 or CD90) using anti-Thy-1 MBs [Citation46]. The latter two are specific endothelial markers for human cancer types, which are a challenge to investigate in a preclinical setting. The Willmann group [Citation45,Citation46] therefore developed a mouse model that expresses human vascular biomarkers by transfecting mouse endothelial cells with the human biomarker of interest and implanting these together with the tumour cells of interest.
In a preclinical setting Targestar-B was targeted to epidermal growth factor receptor (EGFR) and CD147 expressed in head and neck squamous cell carcinoma, where using the dual targeting was reported as most promising [Citation47]. Although E-selectin is not a novel target, its up-regulation due to inflammation can also be used to monitor tumour progression and metastasis. E-selectin can therefore be used as a new targeting strategy for early screening for tumours with metastatic potential [Citation26]. Next to novel targeting strategies for tumours, ischaemia-reperfusion after myocardial infarction was targeted to matrix metalloproteinase-2 (MMP-2) using polymer-shelled microcapsules. Apoptosis is another recently studied target, typically targeted to PS. Annexin V is known to specifically bind to PS [Citation48] and was used for apoptosis imaging in breast cancer cells using nanobubbles [Citation24].
Techniques to enhance binding
Binding of tUCAs to target biomarkers under in vivo conditions is a key factor for successful application of molecular imaging for diagnosis and therapy (). Methods to enhance binding of targeted MBs (tMBs) generally depend on adjustments in the coating of the tMBs and/or applying acoustic radiation force to push the tMBs to the target cells. Deflating the tMBs to increase the shell surface area [Citation36], conjugating two [Citation49] or three [Citation50] different ligands to the coating, or optimising the length of the ligand linker [Citation51] are among those studies optimising the tMBs for an enhanced binding effect. Also, the probability of successful binding may be increased by homogenising the ligand distribution on the MB shell [Citation13], but needs further investigation. In addition, larger tMBs have a higher binding force because of their larger binding area. Nevertheless, the shear forces induced by the blood flow experienced by larger tMBs are higher than those experienced by smaller tMBs; this increases the risk of detachment of larger tMBs from their targets. In a numerical study, the optimal tMB size for enhanced binding (assuming they keep their spherical shape) is suggested to be in the range of 2–4 µm in diameter [Citation52]. In a very recent study, super-resolution microscopy was used to compare tMBs based on DSPC or DPPC in terms of ligand distribution, binding area, and their shape upon binding () [Citation13]. This study shows that DSPC tMBs keep their spherical shapes after binding () and have significantly smaller binding areas than DPPC tMBs, which had a dome shape after binding (). Magnetic MBs have also been developed to enhance the targeting in UMI and therapy [Citation53–55]. Magnetic targeting uses an externally applied magnetic field, typically applied using a permanent magnet, to control the location of magnetically responsive MBs. Various magnetic MB preparations and applications have been published by Stride et al. in 2009 and 2012 [Citation54,Citation56]. For instance, the gas core of the MB can be surrounded by a ferrofluid and stabilised by an outer coating of L-α-PC. Such a magnetic MB could increase the dwell time of tMBs in a target volume, whilst specificity could be provided by biochemical targeting [Citation56].
In addition to the tMB shell modifications, acoustic radiation force [Citation57] can be used to push the MBs against the vessel wall to further improve the targeting rate of the MBs [Citation58–61]. This technique was also successfully used to improve the delivery of stem cells to the vessel wall which can be used for the repair of damaged tissue () by developing echogenic complexes by conjugating tMBs to stem cells (StemBells) [Citation62].
Measuring binding force
To evaluate the efficacy and strength of tUCAs, a measurement system capable of assessing the strength of various binding configurations is necessary. Several in vitro methods have been proposed. Kim et al. [Citation63] used a micromanipulation technique to adhere a single tMB to an individual glass bead using two separate pipettes (). The pressure applied by the bead pipette was incrementally increased until the MB detached from the bead. Using this method, the binding force was measured as a function of composition and structure of the lipid shell and the receptor-ligand pair in a controlled in vitro environment. For instance, the detachment force for the biotin-PEG-avidin system was measured to be in the order of 100 nN assuming the contact area of the MBs and the coated surface to be around 10 µm2. In another study, atomic force microscopy (AFM) was used for assessing the adhesive interactions of tMBs with their target cells in vitro [Citation64]. They used in-house developed lipid-shelled tMBs conjugated to the CD31 antibody using biotin-avidin bridging for adhesion to SK-HEP-1 hepatic endothelial cells and measured single distributions of the binding forces with a median of 93 pN. Controlled shear flow has also shown potential for monitoring the binding force of MBs targeted to P-selectin in vitro [Citation65]. In this experiment attachment and detachment of tMBs to P-selectin immobilised on a culture dish was investigated in a parallel-plate flow chamber by increasing shear stress at intervals of 30 s. The accumulation rate first increased with shear stress, reached a maximum at ∼0.6 dyn/cm2 and then decreased. Half-maximal detachment was reached at 34 dyn/cm2. These results suggest that accumulation and retention of tMBs are possible under physiological flow conditions [Citation39]. Another approach for measuring binding force is the effect of secondary Bjerknes forces on tMBs, which was studied using a high-speed camera [Citation66,Citation67]. The secondary Bjerknes force is an averaged net force that neighbouring MBs experience due to their oscillations in an ultrasound field. The direction of the force depends on the phase difference between the MB oscillations and the oscillating pressure gradient [Citation68]. Kokhuis et al. [Citation66] observed that bound tMBs deform in the direction of their neighbouring MB when they were subjected to secondary Bjerknes forces. If low intensity ultrasound is applied, the deformation induces an elastic restoring force, causing the MBs to recoil back to their equilibrium position. For higher acoustic pressures, the secondary Bjerknes force can break the bond between the tMB and the surface. Using this technique, the binding force between a single biotinylated MB and an avidin-coated surface was measured to be between 0.9 and 2 nN. In addition, the optical observation of the event suggests that lipid anchors are pulled out of the MBs shell, rather than destruction of the strong bond between biotin and avidin [Citation66].
Characterisation of tUCA
Non-targeted MBs for contrast-enhanced imaging, such as SonoVue and Definity, have been thoroughly characterised in terms of their acoustic behaviour in an US field [Citation69–72]. These studies mainly focused on bulk measurements, as regular contrast-enhanced US imaging is also performed in vessels containing high concentrations of MBs. However, for imaging of tMBs where only very few adhered MBs may be in the imaging field, the response of only a single MB or a cluster of MBs has to be detected [Citation73]. The response of a single MB in an US field is therefore of high interest to aid enhancement of the backscattered signal to improve imaging. A first step is to determine the MB properties after attaching a targeting ligand to it. The next step is the characterisation of tMBs adhered to their molecular targets, and comparing this to the response of non-adhered tMBs to find parameters to distinguish them from each other.
Functionalised lipid-coated MBs
Only a few studies used functionalised MBs to determine the effect of functionalisation on the MB properties; in particular their elasticity using either atomic force microscopy (AFM) or high-speed optical imaging. Using AFM, an elasticity was found that was almost 30 × higher for streptavidin-functionalised bubbles than for bare lipid-coated bubbles (710 ± 41 vs. 25 ± 1.4 mN/m; DPPC:PE-biotin, 90:10 mol%) [Citation74]. Recently, the same group performed AFM studies on bubbles with a C3F8 core and a lipid-coating of DPPC:DSPE-PEG(2000)-biotin or DPPC:DOPE-biotin (1,2-dioleoyl-sn-glycero-3-phosphoethanolamine-biotin) in a 90:10 ratio [Citation75]. Both types were functionalised with streptavidin and bubbles with diameters between 3 and 4 μm were deformed up to 20% of their original size, whereas non-functionalised bubbles were deformed up to 50%. Here, streptavidin functionalisation increased the elasticity to 26.9 ± 1.4 mN/m for the PEGylated bubbles, but PEGylation itself was also found to increase the elasticity (17.7 ± 0.7 vs. 10.7 ± 0.5 mN/m). However, the increase in elasticity between PEGylated and non-PEGylated MBs might actually be a consequence of the different lipids that were used for both bubble types: DSPE or DOPE as it has recently been shown that the main lipid of non-targeted biotinylated MBs changes the distribution of the lipids in the shell and its shell properties [Citation13,Citation14]. The authors do not explain the large difference in stiffness values between both studies [Citation74,Citation75]. Indeed, there does not seem to be a straightforward explanation. The preparation method is identical, but although the biotinylated lipids are different this cannot explain such a large difference. The major difference between both studies is the PEGylation [Citation75], but this was found to increase the stiffness, and contradicts with their results.
To study the influence of functionalisation on several MB properties, we functionalised identical biotinylated MBs – with either DSPC or DPPC as the main coating lipid [Citation13] – with streptavidin via avidin-biotin bridging [Citation76]. These unbound bubbles were investigated at frequencies between 1 and 4 MHz at a pressure of 50 kPa and their vibrational response was recorded by optical ultrahigh-speed imaging [Citation14,Citation77]. For DSPC- and DPPC-based bubbles the acoustic stability increased after functionalisation, although their shrinkage remained significant (). The resonance frequencies of functionalised and non-functionalised DSPC MBs were similar, whereas those of DPPC MBs were higher for the functionalised ones. The number of MBs responsive at the subharmonic (SH) frequency was slightly lower for functionalised DSPC bubbles than for non-functionalised DSPC bubbles. For DPPC there was no change after functionalisation. At the second harmonic frequency the functionalised and non-functionalised DSPC bubbles behaved similarly, whereas after functionalisation hardly any DPPC bubbles responded. The viscoelastic shell properties of both functionalised and non-functionalised MBs were estimated [Citation14,Citation77] using the Marmottant model [Citation78]. The shell elasticity for DSPC slightly increased after functionalisation, whereas for DPPC the elasticity increased almost four-fold. The shell damping and viscosity, on the other hand, did not change after functionalisation.
Table 1. Characteristics of streptavidin-functionalised and non-functionalised DSPC and DPPC bubbles.
A critical side note regarding the elasticity estimated for the functionalised DPPC MBs is the narrow size distribution that did not cover the same range as the DSPC bubbles (DPPC 4.8–6.2 μm; DSPC 3.1–7.3 μm). However, our results clearly show an increase of the resonance frequency and thus of the elasticity, which corresponds with the results obtained using AFM [Citation74,Citation75]. The increase in elasticity after functionalisation is believed to be due to the presence of crystallisation of streptavidin around the lipid shell forming a stiffer external layer [Citation75] as also observed on streptavidin-functionalised giant unilamellar phospholipid vesicles [Citation79,Citation80]. This is also consistent with the increased stability; a stiffer and thicker shell better prevents gas escape [Citation81]. The above mentioned studies all found differences between streptavidin-functionalised MBs and non-functionalised MBs. In contrast, Overvelde et al. [Citation82] concluded that the frequency of maximum response and the maximum amplitude of oscillation of functionalised bubbles (BG-6438, Bracco Imaging) and non-functionalised bubbles (BG-6437, Bracco) did not seem to change.
Although streptavidin-biotin binding is a useful tool to gain insights into the effects of coupling of relatively large and heavy ligands to MBs in vitro, it can never be used clinically due to strong immune responses [Citation23]. Therefore, alternatives for in vivo targeting have to be considered, such as peptides, polymers, or antibodies, as discussed in the Targeting and binding section above. Peptides consist of a few amino acids (∼100–200 Da) [Citation83] and the RGD-peptide used typically for targeting has a molecular mass of ∼380 Da. Since functionalising bubbles with a small, low molecular mass ligand such as biotin (∼250 Da) has hardly any effect on the MB response, it is expected that functionalisation of MBs with a peptide also has minimal effect. Polymers are regularly used in MB designs to increase circulation times and to function as a stealth mechanism, mostly in the form of a PEG-lipid [Citation84]. Abou-Saleh et al. [Citation75] only mentioned a slight increase in elasticity upon PEGylation, but it is not known whether addition of a polymer changes other properties. Streptavidin-functionalisation, on the other hand, has a significant effect on MB response. Since antibodies (∼150 kDa) are more than 2 × heavier than streptavidin (∼60 kDa) an even larger effect, especially on the elasticity and resonance frequency, is expected. In addition, coupling an antibody covalently to a lipid could also induce cross-linking of the lipids as there are usually several reactive groups on an antibody.
Discriminating free from adhered MBs
The next step after functionalisation of MBs is studying their behaviour when they have bound to their molecular target. Moreover, the differences in acoustical signals between free and adhered bubbles are the key feature to facilitate discrimination.
Some of the non-functionalised biotinylated bubble types described in the previous section [Citation13,Citation14,Citation73] were used to target an avidin-coated cellulose capillary using acoustic radiation force to promote binding [Citation85]. Optical imaging was used to confirm bubble adhesion, and high-speed optical imaging was used to visualise the oscillations [Citation86]. The adherent MBs oscillated symmetrically in the plane parallel to the wall (similar to a free-floating MB) and asymmetrically in the plane normal to the wall. The side of the MB near the boundary expanded and contracted to a lesser extent than the side away from the boundary. The normalised radial expansion was larger for adherent bubbles in both imaging planes. When the transmission pressure was increased from 240 to 450 kPa, the centre of the MB began to collapse towards the fixed boundary, producing a jet. At even higher pressures (650 kPa) fragmentation was observed in the plane parallel to the boundary, where the remaining fragments expanded and contracted, and were displaced along the wall away from the ultrasound source [Citation85]. This can be advantageous for drug delivery applications when the drug is incorporated in or attached to the shell of the MB. The response at the fundamental frequency was larger for adherent bubbles than for free bubbles [Citation86], the responses at the SH frequency were similar, and the second harmonic component also increased [Citation85]. At increasing pressures the signals at the third and fourth harmonic frequencies were also higher for adhered MBs. One of the underlying causes of the higher fundamental (and maybe also harmonic) signals of adherent bubbles could be due to the small diameter of the bubbles (<< acoustic wavelength) and the nearly uniform spatial distribution of free bubbles, resulting in incoherent echo summation and a small backscattered intensity from each sample volume. Alternatively, a layer of tUCA adherent to the inside of a vessel wall reflects US coherently, resulting in a large reflection of the fundamental component. Secondly, the adherent bubbles formed aggregates, which increase the coupling between adjacent bubbles and thus their effective scattering cross section [Citation86].
In addition to adhered and free floating MBs, Overvelde et al. [Citation82] studied bubbles close to an OptiCell wall, using ultrahigh-speed imaging. For non-functionalised bubbles close to the wall, the amplitudes at the frequency of maximum response were lower than for free-floating non-functionalised MBs. However, the OptiCell wall was not blocked for unspecific binding, hence these bubbles probably also adhered to the wall and cannot be considered as non-adherent. The observation that still holds is the 50% lower frequency of maximum response for adherent functionalised MBs than for functionalised and non-functionalised MBs in the unbounded fluid (150 μm away from the wall). The lower frequency of maximum response for bubbles bound to the OptiCell wall might be due to an increase in damping due to the coupling of the bubble and the wall.
Besides optical interrogation of bubbles, acoustical measurements have been used to characterise tMBs. Prior to the acoustical measurements, the biotinylated bubbles were sized by optical microscopy and subsequently injected in a capillary with or without streptavidin-coating [Citation87]. Scattering of non-biotinylated bubbles and biotinylated bubbles at the fundamental frequency (2 MHz) was similar, whether or not the capillary was coated. The second harmonic resonance radius of an adherent MB was higher than that of a non-adherent targeted bubble, i.e. the second harmonic resonance frequency was higher. The reason that a difference between biotinylated and non-biotinylated bubbles was not found might be just a size or mass effect of the functionalisation ligand. Not only is biotin (∼250 Da) much smaller than streptavidin (∼60 kDa), but one streptavidin molecule can bind up to four biotin molecules [Citation84] that could form a protein layer around the shell, as suggested by others [Citation75,Citation80]. The effect of biotinylation on the resonance frequency might therefore be only minimal.
The most recent study that compared free with bound MBs focused on the SH response frequencies at 11 and 25 MHz [Citation88]. The rationale behind applying higher frequencies is imaging in a preclinical setting, but also these high frequencies are needed in a clinical setting for the assessment of atherosclerosis in the carotid or for superficial tumours. This study used the commercially available Target-ready MicroMarker (lipid-coated and streptavidin functionalised) which had stronger SH activity for larger bubbles when insonified at 11 MHz, i.e. the SH resonance frequency decreased upon binding. At 25 MHz the difference was smaller between free and bound MBs, but the amplitude of the adhered bubbles was 20% higher. In general, the pressure thresholds for SHs were lower at 11 MHz than at 25 MHz. Bound bubbles disrupted at lower pressure thresholds than unbound, especially at 11 MHz. At this frequency mainly compression-only behaviour was observed for both bound and unbound MBs, whereas at 25 MHz the oscillations were expansion-dominated. Although differences in SH resonance were present, no shift in the fundamental resonance frequency was observed. This study also aimed to find a strategy to enhance the SH signal for imaging. Optimal pulse-inversion techniques require the same phase (0 radian phase shift) of the responses induced by both transmit pulses. Consequently, due to complete constructive interference (much like an opposite phase of π radian is desirable between fundamental echoes to ensure complete destructive interference) this would yield the maximal SH amplitude. At 11 MHz the SH emissions were consistently half a wavelength (π/2 radian) out of phase and at 25 MHz it varied more, but a similar trend was observed. This suggests that with the incorporation of a phase-shifting strategy SH signal amplitudes from pulse-inversion techniques can be increased to 60% to enhance imaging.
Interestingly, the above-mentioned studies show an opposite effect on the resonance frequency upon binding of the MBs. For streptavidin-functionalised MBs in an OptiCell insonified at pressures between 2 and 4 MHz, the resonance frequency decreased [Citation82], while for biotinylated MBs in a streptavidin-coated capillary insonified at 2 and 3.5 MHz the resonance frequency increased [Citation87]. At higher frequencies streptavidin-coated bubbles at 11 MHz showed an increase in resonance frequency, while they showed a decrease at 25 MHz [Citation88]. A potential explanation for the opposing trends observed may be frequency-dependent boundary effects, for example caused by frequency-dependent boundary stiffness [Citation88]. Nevertheless, these contradictions show that much is still unknown concerning the effects of binding on the bubble response. As this is the key factor for acoustic differentiation between bound and unbound MBs, single MB studies are needed for reliable determination of the bubble properties. Especially in vivo studies could aid the understanding of MB behaviour in a clinically translatable environment.
Other types of targeted tUCA
Next to the lipid-coated MBs described above, the characteristics of other types of tUCAs such as polymer and nanobubbles have been studied. Schmidt et al. [Citation89] used poly(l-lactic acid) (PLA) capsules that bonded to a neutravidin-adsorbed polystyrene surface (similar to an OptiCell wall). In this study the microcapsules adhered to the surface under flow conditions with wall shear stresses between 0.2 dyn/cm2 and 1.5 dyn/cm2. The capsules first slowed down before binding and were easier to detach at higher shear stresses and higher acoustic pressures. At the highest pressure of 291 kPa an appreciable fraction of the capsules also ruptured and released their gas content.
Recently, polyvinyl alcohol (PVA) capsules gained specific interest due to their chemical versatility that enables functionalisation with different ligands, for instance hyaluronic acid for the targeting of tumour cells and tissues [Citation90]. The properties of the air-filled PVA capsules were compared to other polymer-coated capsules and commercially available bubbles with lipid and protein coatings [Citation91]. At very high pressures up to 2.344 MPa (mechanical index (MI) = 1.58) the shell’s shear modulus was estimated to be 3.7 MPa. Assuming a shell thickness of 0.5 µm [Citation91] this corresponds to an elasticity parameter of 5.6 N/m, an order of magnitude higher than for lipid-coated bubbles [Citation71,Citation72,Citation77]. The in vitro capsule concentration necessary to obtain the same signal was similar to Albunex, 5 to 10-fold lower than for SonoVue and Definity and even 35-fold lower than for Optison. Using the same concentrations for SonoVue and PVA bubbles, the second and higher harmonic signals for PVA were up to 10 dB higher.
Sub-micron sized nanobubbles (∼200 nm) may potentially extravasate by passing the capillary barrier to reach cells at the tumour cell target site [Citation8]. This property makes them promising for targeted molecular imaging and drug delivery in tumours. Due to the higher permeability of tumour vasculature – enhanced permeability and retention (EPR) effect – nanobubbles are more likely to accumulate in tumours, known as passive targeting. The stability of the lipid-Pluronic nanobubbles was higher than that of Definity (in vitro). In mice, the lipid-Pluronic nanobubbles were imaged using contrast harmonic imaging at 8 MHz and their contrast in the tumour was higher than for Definity. This was ascribed to the possible extravasation of the nanobubbles, which retains them in the tumour and thus increased the signal.
Ultrasound molecular imaging
UMI that uses tUCAs is a multidisciplinary technology applicable for both diagnosis, monitoring of lesion formation, and therapy evaluation.
Contrast-specific imaging techniques
MBs generate higher harmonics, SHs and ultraharmonics of the excitation frequency [Citation92–97]. Upon excitation by multi-frequency bursts, MBs can also act as non-linear mixers of the excitation frequencies and produce cross-products [Citation98–100]. Conventional non-linear imaging techniques, at lower frequencies (<15 MHz), focus mainly on detection of higher harmonics [Citation101–105]. The need for high resolution UMI in small animal applications has pushed the frequencies used in preclinical imaging to above 15 MHz [Citation106]. At these frequencies similar non-linear techniques have been implemented [Citation107–111]. However, performance of these imaging methods is degraded because the excitation frequency is much higher than the resonance frequency of the MBs, attenuation is higher, and far-wall artefacts are a big challenge to overcome [Citation112–116]. Therefore, improved imaging methods have been extensively studied, such as improved harmonic imaging methods [Citation117–119], chirp coded excitation alone [Citation120,Citation121] or combined with pulse inversion [Citation122,Citation123]. Among the non-linear components of the MB response the SH signal has drawn much attention lately, due to its MB specificity and artefact-free characteristics. Moreover, SH response of the MB has shown its potential for selectively imaging bound tMBs [Citation124,Citation125]. The SH signal is strongly dependent on the applied acoustic pressures, the ambient pressure variations [Citation93,Citation126–130], and the envelope of the excitation signal [Citation131–135]. The SH signal is also less attenuated than the ultraharmonic and higher harmonics, and therefore a more suitable choice for high frequency applications. Next to the different strategies to improve the sensitivity of MB detection, adjusting the MBs is another approach to gain sensitivity. It has been shown that UMI of sorted 3 -µm MBs results in an approximately 20 times higher video intensity than for unsorted populations [Citation136]. This size lies within the optimal tMB size distribution for enhanced binding, as shown numerically [Citation52]. This can significantly maximise the sensitivity to small numbers of MBs for UMI.
Selective imaging of true bound tMBs from free-flowing unbound ones is another challenge that is extensively studied [Citation137–141]. The most common approach for imaging and quantification of tMBs is to wait for a few minutes (2 [Citation142] to 20 min [Citation143]) so most of the circulating MBs have been taken up by the lungs and liver (i.e. RES system), or have been dissolved. This time also allows the tMBs to accumulate at the site of their targets. Then low power non-destructive pulses are applied to image the tMBs, followed by a high power disruptive pulse (flash) to eliminate the MBs within the imaging plane which is again followed by low power pulses to image the residual circulating MBs (). The intensity difference before and after the flash corresponds to the amount of bound tMBs and is a measure for the biomarker concentration [Citation144–147], as shown in . In such methods the quantification of bound tMBs strongly depends on the injected dose, imaging system gain, and local perfusion [Citation137]. In addition, the influence of inhaled gases in the anaesthetic protocols influences the MBs longevity [Citation148–151]. These studies confirmed longer circulation times of in-house lipid-shell decafluorobutane-filled UCAs and commercially available UCAs such as Definity and Albunex when animals breathe medical air instead of pure oxygen as the carrier gas for the isoflurane anaesthetic. This is perhaps due to a reduced ventilation/perfusion mismatch and classical diffusion between the blood gases and the gas inside the MBs (e.g. perfluorobutane), in which nitrogen plays a role by increasing the volume of the MBs and diluting other gas species in the MBs gas core [Citation151].
Figure 2. Ultrasound molecular imaging. (A) Timeline of the imaging protocol and schematic representation of a typical time intensity curve in the region of interest (e.g. tumour). (B) B-mode (grey) overlaid with non-linear contrast mode (green) US imaging in 3D to detect the αvβ3 expression via αvβ3-tMBs (MicroMarker) adhered to the SVEC cells (SV40-transformed murine endothelial cell line), which were cultured in an OptiCell. The band in the middle of the figure in which there is no green signal present shows the destruction of the tMBs with the flash burst. (C–E) 3D micro-UMI using VEGFR-2 Target-Ready MicroMarker on a subcutaneous human hepatocellular carcinoma tumour which was developed by injection of HuH7 cells in male nude NMRI mice. All animal work was approved by the regulatory authority of Erasmus MC and performed in compliance with the Dutch government regulation guidelines. (C) 3D B-mode US render of the tumour. (D) 3D render of contrast images 10 min after bound tMBs within the entire volume of the tumour. (E) 3D render of contrast images 10 min after injecting the control MBs. Lack of signals within the tumour indicates no attachment of control MBs to the VEGFR-2 receptors. For (B–E), imaging was performed with a Vevo 2100 US imaging system and MS250 probe at 18 MHz. The probe was moved with increments of 32 µm using a step motor (VisualSonics).
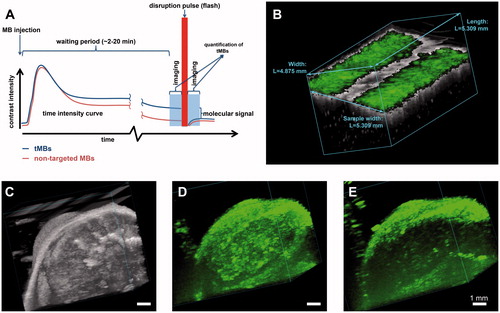
The presence of tissue motion can compromise quantification, as well as high concentrations of freely recirculating MBs after the waiting period. Several methods for selectively imaging the bound tMBs in real time have been proposed: utilising an image–push–image sequence [Citation137], transmission at a low frequency and reception at a high frequency [Citation138], using the SH response of the MBs and interframe filtering [Citation139], and using singular value spectra properties [Citation140]. However, none of the proposed methods have yet been applied in vivo. Only Pysz et al. [Citation152] developed a quantification method based on dwell time MB signal measurements, which was tested in vivo in well vascularised tumours in mice. However, in this in vivo model where attachment of tMBs is significant, the classical way of quantification also performs well. Thus, the performance of the method developed by Pysz et al. in applications with very few tMBs in the presence of circulating MBs remains unclear. Daeichin et al. have developed an off-line quantification method for the detection of biomarker concentrations in vivo in cases with a high number of bound MBs, as well as only very few bound MBs [Citation153]. This method benefits from motion compensation and individual contrast spot detection, and is capable of distinguishing bound MBs from unbound MBs based on their displacement. Such a quantification method can be applied in studies performed with different imaging settings because it is less sensitive to imaging parameters.
In vivo molecular imaging
As discussed previously, the diagnostic focus of UMI is mainly on inflammation, thrombosis and angiogenesis. Assessment of angiogenesis is perhaps the application where UMI is used the most [Citation27,Citation31–34,Citation154–159]. For evaluating tumour growth non-invasively, successful in vivo quantification of the expression levels of the angiogenetic biomarkers αvβ3 integrin, endoglin, and VEGFR2, which vary during tumour growth in subcutaneous cancer xenografts, have been reported [Citation158]. Recently, an UMI study using MBs targeted to αvβ3 in an ovarian cancer model in hens [Citation160], suggested that the detection of ovarian tumour-associated angiogenic microvessels improved when using UMI. For assessing the efficacy of cell-based therapies, UMI has been used to image a genetically engineered cell-surface marker on endothelial progenitor cells to track the fate of these progenitor cells after their delivery into vascular engraftment in vivo within Matrigel plugs [Citation161]. Next to the basic research that is performed using UMI, numerous studies are making steps forward by investigating the possibilities of clinically translatable targeted MBs. In humans, the first phase 0 clinical trial for prostate cancer UMI was presented recently using BR55, a VEGFR2-tUCA [Citation30]. Although it was only a safety study it was reported that 12 out of 14 lesions (proven by histology) could be detected with UMI. Bachawal et al. [Citation162] used BR55 in transgenic mice with breast cancer and ductal carcinoma. UMI allowed for highly accurate detection of both breast cancer and ductal carcinoma in situ and this can be a promising clinical approach for early breast cancer detection. In addition, BR55 has shown its potential for early detection of liver dysplasia in transgenic mice [Citation163].
UMI has also been used to non-invasively assess the effects of anti-inflammatory treatment on endothelial inflammation in early atherosclerosis in genetically modified mice [Citation164,Citation165]. UMI has also proven its ability to detect biomarkers of early response to chemotherapy in several cancer types by MBs targeted to single biomarker expressions (VEGFR2, αVβ3, endoglin, Annexin V, or VEGF-VEGFR complex) [Citation24,Citation166–169] and MBs targeted to two or more of these biomarker expressions [Citation142]. Impressively, studies have shown that UMI using αvβ3-tMBs is a consistent method that can classify a tumour as a responder or a non-responder as early as two days after treatment [Citation170,Citation171]. To establish the link to clinical oncology Flisikowska et al. [Citation172] have suggested the use of larger animal cancer models with more similarities to humans, such as genetically modified pigs. They proposed programmes to generate gene-targeted pigs with mutations in tumour suppressor genes and proto-oncogenes that replicate key lesions responsible for a variety of human cancers. Whilst tumour models in large animals are challenging, cardiovascular disease can more easily be modelled. A study on miniswine suffering from atherosclerosis showed that an improved endothelial permeability through ultrasound-activated nitric oxide-loaded echogenic immunoliposomes, can facilitate the delivery of anti-ICAM-1 conjugated echogenic immunoliposomes to inflammatory components in the arterial wall. This approach therefore has the potential to improve UMI of atheroma [Citation173]. Next to pigs, the expression of P-selectin and VCAM-1 expression in the carotid of non-human primates has also been recently assessed with UMI. This study shows that endothelial cell adhesion molecule expression in large arteries could be an early event that coincides with diet-induced obesity and insulin resistance in non-human primates [Citation174]. In another recent study on non-human primates with myocardial ischaemia, UMI was shown to be both safe and effective for imaging recent myocardial ischaemia. Lipid-coated MBs functionalised with dimeric recombinant human P-selectin glycoprotein ligand-1, a recombinant ligand appropriate for humans, were used [Citation175]. The study suggests that UMI can be useful for detecting recent ischaemia in patients with chest pain, even in the absence of necrosis [Citation175].
Three dimensional UMI
It is challenging to image the same plane repeatedly in 2D UMI serial studies, and small misalignments can already introduce a substantial error. tMBs are attached to their targets and are therefore stationary in UMI applications where tissue motion is absent. This thus opens up opportunities for 3D UMI using 2D probes, by mechanically moving the ultrasound probe over the target of interest. This is illustrated in where 3D UMI is performed with VEGFR2 Target-Ready MicroMarker (FUJIFILM VisualSonics, Inc., Toronto, Ontario, Canada) on subcutaneous human hepatocellular carcinoma tumour in a mouse.
Feasibility of 3D UMI has also been proven by other groups. Streeter et al. [Citation176] performed 3D UMI of tumours expressing αvβ3 integrin by mechanically stepping the transducer across the tumour in 800 µm increments. In another study it was shown that multiple injections of tMBs did not block sufficient binding sites to bias molecular imaging data in serial studies [Citation177], which is an important finding. Using the clinically promising BR55 agent, 3D UMI was shown to be very well suited in depicting the angiogenic activity in very small breast lesions, suggesting its potential for detecting and characterising these lesions at very early stages [Citation178]. Combining the effort to selectively image bound tMBs and 3D UMI, Hu et al. [Citation179] used a broadband single pulse imaging sequence (transmitting at low frequencies and receiving at high frequencies) that is faster than the multi-pulse methods. Then, this method was combined with interframe filtering to selectively image targeted MBs without waiting for clearance of unbound MBs, thereby reducing acquisition time from 10 to 2 min. Their results suggest a feasible method for 3D UMI that is faster than current multi-pulse strategies.
tUCA as theranostics
Enhanced drug delivery
In combination with ultrasound, UCAs are known to enhance drug delivery. Despite the fact that the mechanism behind this enhancement is not well known, vibrating MBs can stimulate drug uptake through cell membrane pore formation, a process also known as sonoporation, opening of intercellular junctions, or endocytosis [Citation6,Citation180]. Whilst UCA-mediated drug delivery has been studied since 1997 [Citation181], tUCA-mediated drug delivery is relatively new. In 2011, Kooiman et al. [Citation10] were the first to show that CD31-tUCAs could sonoporate primary endothelial cells in vitro. Cell membrane permeability () was already induced at acoustic pressures as low as 80–200 kPa (1 MHz, 6 × 10 cycle bursts), indicated by uptake of the co-administered model drug propidium iodide (PI). Since then, several other studies reported tUCAs for drug delivery by either co-administering the drug with the tUCAs or loading the drug in/on the tUCAs. Another co-administration in vitro study using PI showed that sonoporation of cancer cells by αvβ6-tUCAs was higher with chirp pulses from 3–7 MHz than with chirp pulses between 1.3 and 3.1 MHz or single frequency insonification at 2.2 or 5 MHz (110 kPa, 10 μs burst, pulse repetition frequency (PRF) 1 kHz, 2 min treatment) [Citation182]. This can be explained by the fact that chirp pulses cover a broader range of resonance frequencies, as MBs with various sizes have different resonance frequencies; chirp pulses are therefore more efficient than single frequency pulses. In the chicken embryo model (in vivo), a single sine-wave burst (1 MHz, 1000 cycles at 150 or 200 kPa) was sufficient for vascular PI uptake using αvβ3-tUCA as shown in [Citation183].
Figure 3. US and targeted microbubble mediated drug delivery. (A) Schematic of co-administration of the drug together with tUCAs. (B) Propidium iodide (PI) uptake in endothelial cells in vitro induced by vibrating CD31-tUCAs; dashed lines indicate cell borders. Left panel: bright field before US; middle panel: fluorescence before US; right panel: fluorescence after US showing PI uptake (reprinted (adapted) from Kooiman et al. [Citation10]. © 2014, with permission from Elsevier). (C) PI uptake in endothelial cells in vivo induced by vibrating αvβ3-tUCAs. Left panel: bright field before US showing a cluster of six tUCAs; middle panel: fluorescence before US showing the DiI-labelled tUCAs; right panel: fluorescence after US showing PI uptake (© 2014 IEEE. Reprinted, with permission, from Skachkov et al. [Citation183]). (D) Delivery of the drug when it is attached to or incorporated in the tUCA shell. (E) P-selectin-tUCAs are five times more efficient than non-targeted UCAs for gene delivery in vivo in a hind limb ischaemia skeletal muscle model. Validation of luciferase reporter plasmid transfection in rats using bioluminescence (left two panels) and imunohistochemistry (right four panels; arrows point to plasmid transfection in endothelial and perivascular cells; reprinted (adapted) from Xie et al. [Citation186], © 2014, with permission from Elsevier).
![Figure 3. US and targeted microbubble mediated drug delivery. (A) Schematic of co-administration of the drug together with tUCAs. (B) Propidium iodide (PI) uptake in endothelial cells in vitro induced by vibrating CD31-tUCAs; dashed lines indicate cell borders. Left panel: bright field before US; middle panel: fluorescence before US; right panel: fluorescence after US showing PI uptake (reprinted (adapted) from Kooiman et al. [Citation10]. © 2014, with permission from Elsevier). (C) PI uptake in endothelial cells in vivo induced by vibrating αvβ3-tUCAs. Left panel: bright field before US showing a cluster of six tUCAs; middle panel: fluorescence before US showing the DiI-labelled tUCAs; right panel: fluorescence after US showing PI uptake (© 2014 IEEE. Reprinted, with permission, from Skachkov et al. [Citation183]). (D) Delivery of the drug when it is attached to or incorporated in the tUCA shell. (E) P-selectin-tUCAs are five times more efficient than non-targeted UCAs for gene delivery in vivo in a hind limb ischaemia skeletal muscle model. Validation of luciferase reporter plasmid transfection in rats using bioluminescence (left two panels) and imunohistochemistry (right four panels; arrows point to plasmid transfection in endothelial and perivascular cells; reprinted (adapted) from Xie et al. [Citation186], © 2014, with permission from Elsevier).](/cms/asset/6fcf7eca-af26-4075-9fd6-7559045180f0/ihyt_a_997809_f0003_c.jpg)
tUCAs can also be loaded with genetic drugs (plasmid DNA, siRNA, mRNA) or drugs for local gene/drug delivery. Philips et al. [Citation184] used VCAM-1-tUCAs loaded with plasmid DNA to transfect smooth muscle cells with the model gene green fluorescent protein (GFP) in vitro at 1 MHz (200–300 kPa, PRF 100 Hz, ∼5 s per cell) and at 1.5 MHz (200 kPa, PRF 8 kHz, ∼5 s per cell). In another study ovarian cancer cells were transfected with wild-type p53 tumour suppressor gene using luteinising hormone-releasing hormone analogue (LHRHa)-tUCA to induce apoptosis (1 MHz, 0.5 W/cm2, 30 s treatment) [Citation185]. Two studies [Citation186,Citation187] showed that tUCAs loaded with luciferase plasmid can transfect vasculature in vivo. Xie et al. [Citation186] used P-selectin tUCAs in a hind limb ischaemia skeletal muscle model (see ; 1.6 MHz, 0.6–1.8 MPa, power Doppler, pulsing interval 5 s, PRF 2.5 kHz for 10 min), whereas Tlaxca et al. [Citation187] used mucosal addressin cell adhesion molecule-1 or VCAM-1-tUCA in a model for Crohn’s disease (1 MHz, 5 W/cm2, 25% duty cycle for 5 min).
Two types of drugs loaded in tUCAs have been reported for cancer treatment. Paclitaxel loaded into tUCAs induced tumour cell apoptosis in vitro in ovarian cancer cells (LHRHa-tUCAs, 0.3 MHz, 0.5 W/cm2, 30 s treatment) [Citation188] and breast cancer cells (LyP-1-tUCA, 1 MHz, 4 W/cm2, 50% duty cycle, 2 min treatment) [Citation189] as well as in vivo (LHRHa-tUCAs, 0.3 MHz, 1 W/cm2, 50% duty cycle, 3 min treatment) [Citation190]. In vivo, the tUCAs were administered intraperitoneally allowing the tUCAs to adhere to the ovarian cancer cells. The other anti-cancer drug loaded into VEGFR2-tUCAs was BCNU (1,3-bis(2-chloroethyl)-1- nitrosourea) for the treatment of glioma brain tumours in vitro (1 MHz, 0.5 MPa, 10,000 cycles, PRF 5 Hz for 1 min treatment at two sites) and in vivo (1 MHz, 0.7 MPa, 10 ms burst, 5% duty cycle, PRF 5 Hz, 1 min treatment at two sites), where in vivo the tUCAs were also used to open the blood–brain barrier [Citation191].
tUCA vs. non-tUCA for enhanced drug delivery
Strikingly, when tUCAs for drug delivery are directly compared with their non-targeted UCA counterparts, tUCAs are more efficient both in vitro [Citation184,Citation185,Citation188,Citation189,Citation192] (up to 7.7-fold higher [Citation189]) and in vivo [Citation186,Citation187,Citation190,Citation191] (up to 5-fold higher [Citation186]), regardless of whether the drug was co-administered with the tUCAs or whether the drug was loaded on/in the tUCAs. Although the reasons for this higher efficiency have not yet been investigated, several could be possible. Possibly the main reason could be that tUCAs vibrate against the cells directly as they are bound to the cells, which may result in a more efficient transfer of acoustic energy, especially because sonoporation was only reported in cells adjacent to vibrating MBs for non-tUCAs [Citation193,Citation194]. The acoustic behaviour of the tUCAs themselves could also explain this difference, as in vitro studies have shown that tUCAs are acoustically more stable, vibrate with a larger amplitude at the fundamental frequency, fragment in the plane parallel to the boundary and have a different resonance frequency (see Characterisation of tUCA section above). Microbubble clustering could also be a phenomenon contributing to the higher efficiency of tUCAs, as a cluster of bubbles is known to behave as one large bubble, which is also associated with a shift in their resonance frequency [Citation195]. This is substantiated by the chicken embryo study in which clusters of 10 to16 tMBs had a 16-fold higher sonoporation efficacy than single tMBs [Citation13].
A disadvantage of using tUCAs for drug delivery was reported by Hu et al. [Citation196] who showed that insonified αvβ3-tUCAs temporarily decreased blood flow within the insonified area after application of a 5 MHz, 2 or 4 MPa colour Doppler destruction pulse (6-cycle pulse length, PRF 124 Hz, 900 ms duration). Although such high frequency and pressure pulses are not typically used for drug delivery, temporarily reducing the blood flow could be advantageous to keep the delivered drug in the treated area. The reduced blood flow could also reduce tumour size by itself as has been reported for non-tUCA [Citation197].
With tUCAs now also being used for drug delivery, the terms ultrasound-mediated targeted drug delivery or ultrasound and MB targeted drug delivery (UMTD) have become confusing. UMTD is used for drug delivery using non-targeted UCA where ‘targeted’ refers to the local application of the ultrasound itself. We therefore suggest banning the UMTD term and use ultrasound and MB mediated drug delivery (UMMD) for non-targeted UCAs and ultrasound and targeted MB mediated drug delivery (UtMMD) for tUCAs instead.
Outlook and conclusions
Recent studies on larger human-like animals such as pigs and non-human primates, as well as the first phase 0 clinical trial for prostate cancer with the VEGFR2 tUCA BR55 show the capability of clinical UMI in the near future. Recent insights in shell coating properties of tUCAs, new strategies for targeting, and the development of nanoscale tUCAs will open up a new range of opportunities and will broaden the spectrum of diseases that can be targeted.
Intrinsic properties of the tUCAs, such as deformability due to low elasticity could be used as an advantage to improve binding. This underlines the necessity of single tMB investigation to further understand their properties. Upon binding, opposite effects on the resonance frequency are reported, which also reflects a change in harmonic frequencies. The increase in their SH amplitude upon binding could potentially be utilised for selective imaging of adhered tMBs, which could speed up the UMI and increase its specificity. Obviously, 3D imaging is desired for UMI applications, either by mechanically moving a 2D probe or using a volumetric 3D probe.
By using tUCAs as local drug delivery systems, intracellular drug uptake can be enhanced several-fold in comparison to non-tUCAs. tUCAs able to carry and deliver a high payload are needed, as is the elucidation of the mechanism by which tUCA stimulate drug uptake. A novel therapeutic use of tUCAs for cancer treatment could be the functionalisation with kinase inhibitors. These molecules have a high affinity for cancer cell receptors and are already approved or in clinical trials as anticancer drugs, such as gefitinib (EGFR), sunitinib (FLT-1), and Bevacizumab (VEGF) [Citation198]. Bound kinase inhibitor tUCAs could therefore facilitate both UMI and act as inhibitor activators for apoptosis. With BR55 now undergoing regulatory approval for clinical use, this will hopefully pave the way for other tUCA with other targets as well. However, for every new tUCA or drug-loaded tUCA, regulatory approval will be needed before clinical use.
tUCAs can also be used as theranostic agent without the addition of a drug or gene, but in combination with high intensity focused ultrasound (HIFU) techniques instead. These techniques utilise high-energy focused ultrasound to locally increase the temperature at the focal point for ablation of tumours. UCAs, as synergists, have become a research topic to improve the efficiency of HIFU treatment [Citation199,Citation200]. In this field, a folate-tUCA (phase transition nanoemulsion) has shown great potential to enhance HIFU ablation of ovarian cancer in vivo [Citation201].
In conclusion, adding to the wide applications of UMI for diagnosis, the therapeutic benefits of this technology also play a major role in its popularity. Detection of diseased cells using tUCAs combined with local drug delivery, sonoporation, and HIFU are good examples of the applications and the potential of UMI for therapy.
Declaration of interest
This research is partly supported by the Center for Translational Molecular Medicine and the Dutch Heart Foundation (PARISk), and by NanoNextNL, a micro and nanotechnology consortium of the Government of the Netherlands and 130 partners. This work is also part of the research programme Veni, which is financed by the Netherlands Organisation for Scientific Research (NWO) (K.K.). The authors alone are responsible for the content and writing of the paper.
References
- Kaul S. Myocardial contrast echocardiography: A 25-year retrospective. Circulation 2008;118:291–308
- Alzaraa A, Gravante G, Chung WY, Al-Leswas D, Morgan B, Dennison A, et al. Contrast-enhanced ultrasound in the preoperative, intraoperative and postoperative assessment of liver lesions. Hepatol Res 2013;43:809–19
- Klibanov AL. Ultrasound contrast materials in cardiovascular medicine: From perfusion assessment to molecular imaging. J Cardiovasc Transl Res 2013;6:729–39
- Correas JM, Helenon O, Pourcelot L, Moreau JF. Ultrasound contrast agents. Examples of blood pool agents. Acta Radiol Suppl 1997;412:101–12
- Greis C. Ultrasound contrast agents as markers of vascularity and microcirculation. Clin Hemorheol Microcirc 2009;43:1–9
- Kooiman K, Vos HJ, Versluis M, de Jong N. Acoustic behavior of microbubbles and implications for drug delivery. Adv Drug Deliv Rev 2014;72:28–48
- Hernot S, Klibanov AL. Microbubbles in ultrasound-triggered drug and gene delivery. Adv Drug Deliv Rev 2008;60:1153–66
- Wu H, Rognin NG, Krupka TM, Solorio L, Yoshiara H, Guenette G, et al. Acoustic characterization and pharmacokinetic analyses of new nanobubble ultrasound contrast agents. Ultrasound Med Biol 2013;39:2137–46
- Wang L, Li L, Guo Y, Tong H, Fan X, Ding J, et al. Construction and in vitro/in vivo targeting of PSMA-targeted nanoscale microbubbles in prostate cancer. Prostate 2013;73:1147–58
- Kooiman K, Foppen-Harteveld M, van der Steen AF, de Jong N. Sonoporation of endothelial cells by vibrating targeted microbubbles. J Control Release 2011;154:35–41
- Alzaraa A, Gravante G, Chung WY, Al-Leswas D, Bruno M, Dennison AR, et al. Targeted microbubbles in the experimental and clinical setting. Am J Surg 2012;204:355–66
- Unnikrishnan S, Klibanov AL. Microbubbles as ultrasound contrast agents for molecular imaging: Preparation and application. Am J Roentgenol 2012;199:292–9
- Kooiman K, Kokhuis TJA, van Rooij T, Skachkov I, Nigg A, Bosch JG, et al. DSPC or DPPC as main shell component influences ligand distribution and binding area of lipid-coated targeted microbubbles. Eur J Lipid Sci Technol 2014;116:1217–27
- van Rooij T, Luan Y, Renaud GGJ, van der Steen AFW, De Jong N, Kooiman K. Acoustical response of DSPC versus DPPC lipid-coated microbubbles. Proc IEEE Ultrason Symp 2013; 2013 July 22–25; Prague, Czech Republic. pp 310–13
- Myrset AH, Fjerdingstad HB, Bendiksen R, Arbo BE, Bjerke RM, Johansen JH, et al. Design and characterization of targeted ultrasound microbubbles for diagnostic use. Ultrasound Med Biol 2011;37:136–50
- Marsh D, Bartucci R, Sportelli L. Lipid membranes with grafted polymers: Physicochemical aspects. Biochim Biophys Acta 2003;2:1–2
- Ottoboni S, Short RE, Kerby MB, Tickner EG, Steadman E, Ottoboni TB. Characterization of the in vitro adherence behavior of ultrasound responsive double-shelled microspheres targeted to cellular adhesion molecules. Contrast Media Mol Imaging 2006;1:279–90
- Chen CC, Borden MA. The role of poly(ethylene glycol) brush architecture in complement activation on targeted microbubble surfaces. Biomaterials 2011;32:6579–87
- Kiessling F, Fokong S, Bzyl J, Lederle W, Palmowski M, Lammers T. Recent advances in molecular, multimodal and theranostic ultrasound imaging. Adv Drug Deliv Rev 2014;72:15–27
- Hernot S, Unnikrishnan S, Du Z, Shevchenko T, Cosyns B, Broisat A, et al. Nanobody-coupled microbubbles as novel molecular tracer. J Control Release 2012;158:346–53
- Nakatsuka MA, Mattrey RF, Esener SC, Cha JN, Goodwin AP. Aptamer-crosslinked microbubbles: Smart contrast agents for thrombin-activated ultrasound imaging. Adv Mater 2012;24:6010–16
- Wheatley MA, Lathia JD, Oum KL. Polymeric ultrasound contrast agents targeted to integrins: Importance of process methods and surface density of ligands. Biomacromolecules 2007;8:516–22
- Paganelli G, Belloni C, Magnani P, Zito F, Pasini A, Sassi I, et al. Two-step tumour targetting in ovarian cancer patients using biotinylated monoclonal antibodies and radioactive streptavidin. Eur J Nucl Med 1992;19:322–9
- Wei X, Li Y, Zhang S, Gao X, Luo Y, Gao M. Ultrasound targeted apoptosis imaging in monitoring early tumor response of trastuzumab in a murine tumor xenograft model of her-2-positive breast cancer. Transl Oncol 2014;7:284–91
- Pochon S, Tardy I, Bussat P, Bettinger T, Brochot J, von Wronski M, et al. BR55: A lipopeptide-based VEGFR2-targeted ultrasound contrast agent for molecular imaging of angiogenesis. Invest Radiol 2010;45:89–95
- Fokong S, Fragoso A, Rix A, Curaj A, Wu Z, Lederle W, et al. Ultrasound molecular imaging of E-selectin in tumor vessels using poly n-butyl cyanoacrylate microbubbles covalently coupled to a short targeting peptide. Invest Radiol 2013;48:843–50
- Willmann JK, Lutz AM, Paulmurugan R, Patel MR, Chu P, Rosenberg J, et al. Dual-targeted contrast agent for US assessment of tumor angiogenesis in vivo. Radiology 2008;248:936–44
- Jun HY, Park SH, Kim HS, Yoon KH. Long residence time of ultrasound microbubbles targeted to integrin in murine tumor model. Acad Radiol 2010;17:54–60
- Otani K, Yamahara K. Feasibility of lactadherin-bearing clinically available microbubbles as ultrasound contrast agent for angiogenesis. Mol Imaging Biol 2013;15:534–41
- Wijkstra HSM, Rosette JJ, de la Pochon S, Tranquart F. Targeted microbubble prostate cancer imaging with BR55. 17th Eur Symp Ultrasound Imaging; 2012 January 1920; Rotterdam, the Netherlands. pp 6–7
- Kaufmann BA, Sanders JM, Davis C, Xie A, Aldred P, Sarembock IJ, et al. Molecular imaging of inflammation in atherosclerosis with targeted ultrasound detection of vascular cell adhesion molecule-1. Circulation 2007;116:276–84
- Kaufmann BA, Lewis C, Xie A, Mirza-Mohd A, Lindner JR. Detection of recent myocardial ischaemia by molecular imaging of P-selectin with targeted contrast echocardiography. Eur Heart J 2007;28:2011–17
- Kim H, Moody MR, Laing ST, Kee PH, Huang SL, Klegerman ME, et al. In vivo volumetric intravascular ultrasound visualization of early/inflammatory arterial atheroma using targeted echogenic immunoliposomes. Invest Radiol 2010;45:685–91
- Kaufmann BA, Carr CL, Belcik JT, Xie A, Yue Q, Chadderdon S, et al. Molecular imaging of the initial inflammatory response in atherosclerosis: Implications for early detection of disease. Arterioscler Thromb Vasc Biol 2010;30:54–9
- Wen Q, Wan S, Liu Z, Xu S, Wang H, Yang B. Ultrasound contrast agents and ultrasound molecular imaging. J Nanosci Nanotechnol 2014;14:190–209
- Rychak JJ, Lindner JR, Ley K, Klibanov AL. Deformable gas-filled microbubbles targeted to P-selectin. J Control Release 2006;114:288–99
- Wang X, Hagemeyer CE, Hohmann JD, Leitner E, Armstrong PC, Jia F, et al. Novel single-chain antibody-targeted microbubbles for molecular ultrasound imaging of thrombosis: Validation of a unique noninvasive method for rapid and sensitive detection of thrombi and monitoring of success or failure of thrombolysis in mice. Circulation 2012;125:3117–26
- Schumann PA, Christiansen JP, Quigley RM, McCreery TP, Sweitzer RH, Unger EC, et al. Targeted-microbubble binding selectively to GPIIb IIIa receptors of platelet thrombi. Invest Radiol 2002;37:587–93
- Cheng C, Helderman F, Tempel D, Segers D, Hierck B, Poelmann R, et al. Large variations in absolute wall shear stress levels within one species and between species. Atherosclerosis 2007;195:225–35
- Nakatsuka MA, Barback CV, Fitch KR, Farwell AR, Esener SC, Mattrey RF, et al. In vivo ultrasound visualization of non-occlusive blood clots with thrombin-sensitive contrast agents. Biomaterials 2013;34:9559–65
- Thompson IM, Pauler DK, Goodman PJ, Tangen CM, Lucia MS, Parnes HL, et al. Prevalence of prostate cancer among men with a prostate-specific antigen level < or = 4.0 ng per milliliter. N Engl J Med 2004;350:2239–46
- Perner S, Hofer MD, Kim R, Shah RB, Li H, Moller P, et al. Prostate-specific membrane antigen expression as a predictor of prostate cancer progression. Hum Pathol 2007;38:696–701
- Sanna V, Pintus G, Bandiera P, Anedda R, Punzoni S, Sanna B, et al. Development of polymeric microbubbles targeted to prostate-specific membrane antigen as prototype of novel ultrasound contrast agents. Mol Pharm 2011;8:748–57
- Tsuruta JK, Klauber-DeMore N, Streeter J, Samples J, Patterson C, Mumper RJ, et al. Ultrasound molecular imaging of secreted frizzled related protein-2 expression in murine angiosarcoma. PLoS One 2014;9:e86642
- Lutz AM, Bachawal SV, Drescher CW, Pysz MA, Willmann JK, Gambhir SS. Ultrasound molecular imaging in a human CD276 expression-modulated murine ovarian cancer model. Clin Cancer Res 2014;20:1313–22
- Foygel K, Wang H, Machtaler S, Lutz AM, Chen R, Pysz M, et al. Detection of pancreatic ductal adenocarcinoma in mice by ultrasound imaging of thymocyte differentiation antigen 1. Gastroenterology 2013;145:885–94 e3
- Knowles JA, Heath CH, Saini R, Umphrey H, Warram J, Hoyt K, et al. Molecular targeting of ultrasonographic contrast agent for detection of head and neck squamous cell carcinoma. Arch Otolaryngol Head Neck Surg 2012;138:662–8
- Koopman G, Reutelingsperger CP, Kuijten GA, Keehnen RM, Pals ST, van Oers MH. Annexin V for flow cytometric detection of phosphatidylserine expression on B cells undergoing apoptosis. Blood 1994;84:1415–20
- Ferrante EA, Pickard JE, Rychak J, Klibanov A, Ley K. Dual targeting improves microbubble contrast agent adhesion to VCAM-1 and P-selectin under flow. J Control Release 2009;140:100–7
- Warram JM, Sorace AG, Saini R, Umphrey HR, Zinn KR, Hoyt K. A triple-targeted ultrasound contrast agent provides improved localization to tumor vasculature. J Ultrasound Med 2011;30:921–31
- Ham AS, Klibanov AL, Lawrence MB. Action at a distance: Lengthening adhesion bonds with poly(ethylene glycol) spacers enhances mechanically stressed affinity for improved vascular targeting of microparticles. Langmuir 2009;25:10038–44
- Maul TM, Dudgeon DD, Beste MT, Hammer DA, Lazo JS, Villanueva FS, et al. Optimization of ultrasound contrast agents with computational models to improve selection of ligands and binding strength. Biotechnol Bioeng 2010;107:854–64
- Kawan S, Hiroshi W. Development of magnetic microbubbles for drug delivery system (DDS). Jpn J Appl Phys 2000;39:S3230–2
- Stride E, Porter C, Prieto AG, Pankhurst Q. Enhancement of microbubble mediated gene delivery by simultaneous exposure to ultrasonic and magnetic fields. Ultrasound Med Biol 2009;35:861–8
- Soetanto K, Watarai H. Ferromagnetic ultrasound microbubbles contrast agent. Engineering in Medicine and Biology Society, 2003 Proc 25th IEEE EMBS Annu Int Conf; 2013 September 17–21; Cancun, Mexico. pp 1226–9
- Owen J, Pankhurst Q, Stride E. Magnetic targeting and ultrasound mediated drug delivery: Benefits, limitations and combination. Int J Hyperthermia 2012;28:362–73
- Torr GR. The acoustic radiation force. Am J Phys 1984;52:402–8
- Borden MA, Sarantos MR, Stieger SM, Simon SI, Ferrara KW, Dayton PA. Ultrasound radiation force modulates ligand availability on targeted contrast agents. Mol Imaging 2006;5:139–47
- Borden MA, Streeter JE, Sirsi SR, Dayton PA. In vivo demonstration of cancer molecular imaging with ultrasound radiation force and buried-ligand microbubbles. Mol Imaging 2013;12:1–8
- Wang S, Hossack JA, Klibanov AL, Mauldin FW. Binding dynamics of targeted microbubbles in response to modulated acoustic radiation force. Phys Med Biol 2014;59:465–84
- Frinking PJ, Tardy I, Theraulaz M, Arditi M, Powers J, Pochon S, et al. Effects of acoustic radiation force on the binding efficiency of BR55, a VEGFR2-specific ultrasound contrast agent. Ultrasound Med Biol 2012;38:1460–9
- Kokhuis TJA, Skachkov I, Naaijkens BA, Juffermans LJM, Kamp O, Kooiman K, et al. Intravital microscopy of localized stem cell delivery using microbubbles and acoustic radiation force. Biotechnol Bioeng 2014;112:220–7
- Kim DH, Klibanov AL, Needham D. The influence of tiered layers of surface-grafted poly(ethylene glycol) on receptor−ligand-mediated adhesion between phospholipid monolayer-stabilized microbubbles and coated glass beads. Langmuir 2000;16:2808–17
- Sboros V, Glynos E, Ross JA, Moran CM, Pye SD, Butler M, et al. Probing microbubble targeting with atomic force microscopy. Colloids and Surfaces B 2010;80:12–17
- Takalkar AM, Klibanov AL, Rychak JJ, Lindner JR, Ley K. Binding and detachment dynamics of microbubbles targeted to P-selectin under controlled shear flow. J Control Release 2004;96:473–82
- Kokhuis TJA, Garbin V, Kooiman K, Naaijkens BA, Juffermans LJM, Kamp O, et al. Secondary Bjerknes forces deform targeted microbubbles. Ultrasound Med Biol 2013;39:490–506
- Garbin V, Cojoc D, Ferrari E, Di Fabrizio E, Overvelde MLJ, van der Meer SM, et al. Changes in microbubble dynamics near a boundary revealed by combined optical micromanipulation and high-speed imaging. Appl Phys Lett 2007;90:114103-1–3
- Leighton TG. The Acoustic Bubble. London: Academic Press, 1994
- Chetty K, Stride E, Sennoga CA, Hajnal JV, Eckersley RJ. High-speed optical observations and simulation results of SonoVue microbubbles at low-pressure insonation. IEEE Trans Ultrason Ferroelectr Freq Control 2008;55:1333–42
- Faez T, Goertz D, De Jong N. Characterization of Definity ultrasound contrast agent at frequency range of 5–15 MHz. Ultrasound Med Biol 2011;37:338–42
- Gorce JM, Arditi M, Schneider M. Influence of bubble size distribution on the echogenicity of ultrasound contrast agents: A study of SonoVue. Invest Radiol 2000;35:661–71
- Helfield BL, Goertz DE. Nonlinear resonance behavior and linear shell estimates for Definity and MicroMarker assessed with acoustic microbubble spectroscopy. J Acoust Soc Am 2013;13:1158–68
- Klibanov AL, Rasche PT, Hughes MS, Wojdyla JK, Galen KP, Wible JH, Jr et al. Detection of individual microbubbles of ultrasound contrast agents: Imaging of free-floating and targeted bubbles. Invest Radiol 2004;39:187–95
- McKendry JE Grant CA, Johnson BRG, Coletta PL, Evans JA, Evans SD. Force spectroscopy of streptavidin conjugated lipid coated microbubbles. Bubble Sci Eng Technol. 2010;2:48–54
- Abou-Saleh RH, Peyman SA, Critchley K, Evans SD, Thomson NH. Nanomechanics of lipid encapsulated microbubbles with functional coatings. Langmuir 2013;29:4096–103
- Lindner JR, Song J, Christiansen J, Klibanov AL, Xu F, Ley K. Ultrasound assessment of inflammation and renal tissue injury with microbubbles targeted to P-selectin. Circulation 2001;104:2107–12
- van der Meer SM, Dollet B, Voormolen MM, Chin CT, Bouakaz A, de Jong N, et al. Microbubble spectroscopy of ultrasound contrast agents. J Acoust Soc Am 2007;121:648–56
- Marmottant P, van der Meer S, Emmer M, Versluis M, de Jong N, Hilgenfeldt S, et al. A model for large amplitude oscillations of coated bubbles accounting for buckling and rupture. J Acoust Soc Am 2005;118:3499–505
- Dieluweit S, Csiszar A, Rubner W, Fleischhauer J, Houben S, Merkel R. Mechanical properties of bare and protein-coated giant unilamellar phospholipid vesicles. A comparative study of micropipet aspiration and atomic force microscopy. Langmuir 2010;26:11041–9
- Darst SA, Ahlers M, Meller PH, Kubalek EW, Blankenburg R, Ribi HO, et al. Two-dimensional crystals of streptavidin on biotinylated lipid layers and their interactions with biotinylated macromolecules. Biophys J 1991;59:387–96
- Borden MA, Kruse DE, Caskey CF, Zhao S, Dayton PA, Ferrara KW. Influence of lipid shell physicochemical properties on ultrasound-induced microbubble destruction. IEEE Trans Ultrason Ferroelectr Freq Control 2005;52:1992–2002
- Overvelde M, Garbin V, Dollet B, de Jong N, Lohse D, Versluis M. Dynamics of coated microbubbles adherent to a wall. Ultrasound Med Biol 2011;37:1500–8
- Voet D, Voet JG. Amino acids. In: Biochemistry. Somerset, NJ, USA: John Wiley and Sons, Inc.; 1995. pp 58–9
- Klibanov AL. Preparation of targeted microbubbles: Ultrasound contrast agents for molecular imaging. Med Biol Eng Comput 2009;47:875–82
- Zhao S, Ferrara KW, Dayton PA. Asymmetric oscillation of adherent targeted ultrasound contrast agents. Appl Phys Lett 2005;87:134103-1–3
- Zhao S, Kruse DE, Ferrara KW, Dayton PA. Acoustic response from adherent targeted contrast agents. J Acoust Soc Am 2006;120:EL63–9
- Casey J, Sennoga C, Mulvana H, Hajnal JV, Tang MX, Eckersley RJ. Single bubble acoustic characterization and stability measurement of adherent microbubbles. Ultrasound Med Biol 2013;39:903–14
- Helfield BL, Cherin E, Foster FS, Goertz DE. The effect of binding on the subharmonic emissions from individual lipid-encapsulated microbubbles at transmit frequencies of 11 and 25 MHz. Ultrasound Med Biol 2013;39:345–59
- Schmidt BJ, Sousa I, van Beek AA, Bohmer MR. Adhesion and ultrasound-induced delivery from monodisperse microbubbles in a parallel plate flow cell. J Control Release 2008;131:19–26
- Cerroni B, Chiessi E, Margheritelli S, Oddo L, Paradossi G. Polymer shelled microparticles for a targeted doxorubicin delivery in cancer therapy. Biomacromolecules 2011;12:593–601
- Grishenkov D, Kari L, Brodin LK, Brismar TB, Paradossi G. In vitro contrast-enhanced ultrasound measurements of capillary microcirculation: comparison between polymer- and phospholipid-shelled microbubbles. Ultrasonics 2011;51:40–8
- de Jong N, Bouakaz A, Frinking P. Basic acoustic properties of microbubbles. Echocardiography 2002;19:229–40
- Eller A, Flynn HG. Generation of subharmonics of order one-half by bubbles in a sound field. J Acoust Soc Am 1969;46:722–7
- Chomas J, Dayton P, May D, Ferrara K. Nondestructive subharmonic imaging. IEEE Trans Ultrason Ferroelectr Freq Control 2002;49:883–92
- Goertz DE, Frijlink ME, Tempel D, Bhagwandas V, Gisolf A, Krams R, et al. Subharmonic contrast intravascular ultrasound for vasa vasorum imaging. Ultrasound Med Biol 2007;33:1859–72
- Needles A, Goertz D, Karshafian R, Cherin E, Brown A, Burns P, et al. High-frequency subharmonic pulsed-wave Doppler and color flow imaging of microbubble contrast agents. Ultrasound Med Biol 2008;34:1139–51
- Wu CY, Tsao J, Chou YH. An ultrasonic microbubble semi-intermodulated imaging technique. Ultrasound Med Biol 2005;31:1199–210
- Deng CX, Lizzi FL, Kalisz A, Rosado A, Silverman RH, Coleman DJ. Study of ultrasonic contrast agents using a dual-frequency band technique. Ultrasound Med Biol 2000;26:819–31
- Shi WT, Forsberg F. Ultrasonic characterization of the nonlinear properties of contrast microbubbles. Ultrasound Med Biol 2000;26:93–104
- Chen S, Kinnick R, Greenleaf JF, Fatemi M. Difference frequency and its harmonic emitted by microbubbles under dual frequency excitation. Ultrasonics 2006;44:Se123–6
- Burns PN, Powers JE, Simpson DH, Brezina A, Kolin A, Chin CT, et al. Harmonic power mode Doppler using microbubble contrast agents: an improved method for small vessel flow imaging. IEEE Proc Ultrason Symp; 31 October – 3 November 1994; Cannes, France. pp 1547–50
- Pi Hsien C, Shun KK, Shih-Jeh W, Levene HB. Second harmonic imaging and harmonic Doppler measurements with Albunex. IEEE Trans Ultrason Ferroelectr Freq Control 1995;42:1020–7
- Simpson DH, Burns PN. Pulse inversion Doppler: A new method for detecting nonlinear echoes from microbubble contrast agents. IEEE Proc Ultrason Symp; 1997 October 5–8; Toronto, Ontario, Canada. pp 1597–600
- de Jong N, Frinking PJA, Bouakaz A, Ten Cate FJ. Detection procedures of ultrasound contrast agents. Ultrasonics 2000;38:87–92
- Deng CX, Lizzi FL. A review of physical phenomena associated with ultrasonic contrast agents and illustrative clinical applications. Ultrasound Med Biol 2002;28:277–86
- Foster FS, Hossack J, Adamson SL. Micro-ultrasound for preclinical imaging. Interface Focus 2011;1:576–601
- Needles A, Arditi M, Rognin NG, Mehi J, Coulthard T, Bilan-Tracey C, et al. Nonlinear contrast imaging with an array-based micro-ultrasound system. Ultrasound Med Biol 2010;36:2097–106
- Lyshchik A, Fleischer AC, Huamani J, Hallahan DE, Brissova M, Gore JC. Molecular imaging of vascular endothelial growth factor receptor 2 expression using targeted contrast-enhanced high-frequency ultrasonography. J Ultrasound Med 2007;26:1575–86
- Rychak J, Graba J, Cheung A, Mystry B, Lindner J, Kerbel R, et al. Microultrasound molecular imaging of vascular endothelial growth factor receptor 2 in a mouse model of tumor angiogenesis. Mol Imaging 2007;6:289–96
- Willmann JK, Paulmurugan R, Chen K, Gheysens O, Rodriguez-Porcel M, Lutz AM, et al. US imaging of tumor angiogenesis with microbubbles targeted to vascular endothelial growth factor receptor type 2 in mice. Radiology 2008;246:508–18
- Moran CM, Watson RJ, Fox KA, McDicken WN. In vitro acoustic characterisation of four intravenous ultrasonic contrast agents at 30 MHz. Ultrasound Med Biol 2002;28:785–91
- ten Kate GL, Renaud GG, Akkus Z, van den Oord SC, ten Cate FJ, Shamdasani V, et al. Far-wall pseudoenhancement during contrast-enhanced ultrasound of the carotid arteries: Clinical description and in vitro reproduction. Ultrasound Med Biol 2012;38:593–600
- Tang M-X, Kamiyama N, Eckersley RJ. Effects of nonlinear propagation in ultrasound contrast agent imaging. Ultrasound Med Biol 2010;36:459–66
- Renaud G, Bosch JG, De Jong N, van der Steen AW, Shamdasani V, Entrekin R. Counter-propagation interaction for contrast-enhanced ultrasound imaging. IEEE Int Ultrason Symp; 2012 October 7–10; Dresden, Germany. pp 1–4
- Yu H, Jang HJ, Kim TK, Khalili K, Williams R, Lueck G, et al. Pseudoenhancement within the local ablation zone of hepatic tumors due to a nonlinear artifact on contrast-enhanced ultrasound. Am J Roentgenol 2010;194:653–9
- Thapar A, Shalhoub J, Averkiou M, Mannaris C, Davies AH, Leen EL. Dose-dependent artifact in the far wall of the carotid artery at dynamic contrast-enhanced US. Radiology 2012;262:672–9
- Hansen R, Angelsen BAJ, Burns PN, Bouakaz A, Borsboom J, Versluis M, et al. Radial modulation imaging. Proc 10th Eur Symp Ultrasound Contrast Imaging; 2005 January 20–21; Rotterdam, the Netherlands. pp 90–1
- Frijlink ME, Goertz DE, Bouakaz A, van der Steen AF. A simulation study on tissue harmonic imaging with a single-element intravascular ultrasound catheter. J Acoust Soc Am 2006;120:1723–31
- Jimenez-Fernandez J. Nonlinear response to ultrasound of encapsulated microbubbles. Ultrasonics 2012;52:784–93
- Park J, Li X, Zhou Q, Shung KK. Combined chirp coded tissue harmonic and fundamental ultrasound imaging for intravascular ultrasound: 20–60 MHz phantom and ex vivo results. Ultrasonics 2013;53:369–76
- Shen CC, Lin CH. Chirp-encoded excitation for dual-frequency ultrasound tissue harmonic imaging. IEEE Trans Ultrason Ferroelectr Freq Control 2012;59:2420–30
- Park J, Huang Y, Chen R, Lee J, Cummins TM, Zhou Q, et al. Pulse inversion chirp coded tissue harmonic imaging (PI-CTHI) of zebrafish heart using high frame rate ultrasound biomicroscopy. Ann Biomed Eng 2013;41:41–52
- Novell A, Sennoga CA, Escoffre JM, Chaline J, Bouakaz A. Evaluation of chirp reversal power modulation sequence for contrast agent imaging. Phys Med Biol 2014;59:5101–17
- Sprague MR, Cherin E, Goertz DE, Foster FS. Nonlinear emission from individual bound microbubbles at high frequencies. Ultrasound Med Biol 2010;36:313–24
- Helfield BL, Cherin E, Foster FS, Goertz DE. The effect of binding on the subharmonic emissions from individual lipid-encapsulated microbubbles at transmit frequencies of 11 and 25 MHz. Ultrasound Med Biol 2013;39:345–59
- Tjötta JN, Tjötta S. An analytical model for the nearfield of a baffled piston transducer. J Acoust Soc Am 1980;68:334–9
- Faez T, Emmer M, Docter M, Sijl J, Versluis M, de Jong N. Characterizing the subharmonic response of phospholipid-coated microbubbles for carotid imaging. Ultrasound Med Biol 2011;37:958–70
- Shankar PM, Krishna PD, Newhouse VL. Advantages of subharmonic over second harmonic backscatter for contrast-to-tissue echo enhancement. Ultrasound Med Biol 1998;24:395–9
- Forsberg F, Liu JB, Shi WT, Furuse J, Shimizu M, Goldberg BB. In vivo pressure estimation using subharmonic contrast microbubble signals: Proof of concept. IEEE Trans Ultrason Ferroelectr Freq Control 2005;52:581–3
- Zhang D, Gong Y, Gong X, Liu Z, Tan K, Zheng H. Enhancement of subharmonic emission from encapsulated microbubbles by using a chirp excitation technique. Phys Med Biol 2007;52:5531–44
- Daeichin V, Faez T, Renaud G, Bosch JG, van der Steen AF, de Jong N. Effect of self-demodulation on the subharmonic response of contrast agent microbubbles. Phys Med Biol 2012;57:3675–91
- Zheng H, Mukdadi O, Kim H, Hertzberg JR, Shandas R. Advantages in using multifrequency excitation of contrast microbubbles for enhancing echo particle image velocimetry techniques: Initial numerical studies using rectangular and triangular waves. Ultrasound Med Biol 2005;31:99–108
- Biagi E, Breschi L, Vannacci E, Masotti L. Subharmonic emissions from microbubbles: Effect of the driving pulse shape. IEEE Trans Ultrason Ferroelectr Freq Control 2006;53:2174–82
- Masotti L, Biagi E, Breschi L, Vannacci E. Study and characterization of subharmonic emissions by using shaped ultrasonic driving pulse. In: André MP, Akiyama I, Andre M, Arnold W, Bamber J, Burov V, et al., editors. Acoustical imaging. Dordrecht, Netherlands: Springer; 2007. pp 307–15
- Zhang D, Xi X, Zhang Z, Gong X, Chen G, Wu J. A dual-frequency excitation technique for enhancing the sub-harmonic emission from encapsulated microbubbles. Phys Med Biol 2009;54:4257–72
- Streeter JE, Gessner R, Miles I, Dayton PA. Improving sensitivity in ultrasound molecular imaging by tailoring contrast agent size distribution: In vivo studies. Mol Imaging 2010;9:87–95
- Zhao S, Kruse DE, Ferrara KW, Dayton PA. Selective imaging of adherent targeted ultrasound contrast agents. Phys Med Biol 2007;52:2055–72
- Hu X, Zheng H, Kruse DE, Sutcliffe P, Stephens DN, Ferrara KW. A sensitive TLRH targeted imaging technique for ultrasonic molecular imaging. IEEE Trans Ultrason Ferroelectr Freq Control 2010;57:305–16
- Needles A, Couture O, Foster F. A method for differentiating targeted microbubbles in real time using subharmonic micro-ultrasound and interframe filtering. Ultrasound Med Biol 2009;35:1564–73
- Mauldin FW, Jr Dhanaliwala AH, Patil AV, Hossack JA. Real-time targeted molecular imaging using singular value spectra properties to isolate the adherent microbubble signal. Phys Med Biol 2012;57:5275–93
- Pysz MA, Gambhir SS, Willmann JK. Molecular imaging: Current status and emerging strategies. Clin Radiol 2010;65:500–16
- Sorace AG, Saini R, Mahoney M, Hoyt K. Molecular ultrasound imaging using a targeted contrast agent for assessing early tumor response to antiangiogenic therapy. J Ultrasound Med 2012;31:1543–50
- Streeter JE, Gessner RC, Tsuruta J, Feingold S, Dayton PA. Assessment of molecular imaging of angiogenesis with three-dimensional ultrasonography. Mol Imaging 2011;10:460–8
- Stieger SM, Dayton PA, Borden MA, Caskey CF, Griffey SM, Wisner ER, et al. Imaging of angiogenesis using Cadence contrast pulse sequencing and targeted contrast agents. Contrast Media Mol Imaging 2008;3:9–18
- Piedra M, Allroggen A, Lindner JR. Molecular imaging with targeted contrast ultrasound. Cerebrovasc Dis 2009;2:66–74
- Dayton PA, Rychak JJ. Molecular ultrasound imaging using microbubble contrast agents. Front Biosci 2007;12:5124–42
- Anderson CR, Hu X, Zhang H, Tlaxca J, Decleves AE, Houghtaling R, et al. Ultrasound molecular imaging of tumor angiogenesis with an integrin targeted microbubble contrast agent. Invest Radiol 2011;46:215–24
- Itani M, Mattrey RF. The effect of inhaled gases on ultrasound contrast agent longevity in vivo. Mol Imaging Biol 2012;14:40–6
- Wible JH, Jr Wojdyla JK, Bales GL, McMullen WN, Geiser EA, Buss DD. Inhaled gases affect the ultrasound contrast produced by Albunex in anesthetized dogs. J Am Soc Echocardiogr 1996;9:442–51
- McDannold N, Zhang Y, Vykhodtseva N. Blood–brain barrier disruption and vascular damage induced by ultrasound bursts combined with microbubbles can be influenced by choice of anesthesia protocol. Ultrasound Med Biol 2011;37:1259–70
- Mullin L, Gessner R, Kwan J, Kaya M, Borden MA, Dayton PA. Effect of anesthesia carrier gas on in vivo circulation times of ultrasound microbubble contrast agents in rats. Contrast Media Mol Imaging 2011;6:126–31
- Pysz MA, Guracar I, Tian L, Willmann JK. Fast microbubble dwell-time based ultrasonic molecular imaging approach for quantification and monitoring of angiogenesis in cancer. Quant Imaging Med Surg 2012;2:68–80
- Daeichin V, Akkus Z, Hoogi A, Bosch JG, Needles A, Kooiman K, et al. Quantification of targeted microbubbles in contrast enhanced ultrasound. IEEE Int Ultrason Symp; 2013 July 21–25; Prague, Czech Republic. pp 1825–8
- Liu H, Wang X, Tan KB, Liu P, Zhuo ZX, Liu Z, et al. Molecular imaging of vulnerable plaques in rabbits using contrast-enhanced ultrasound targeting to vascular endothelial growth factor receptor-2. J Clin Ultrasound 2011;39:83–90
- Hamilton A, Huang SL, Warnick D, Stein A, Rabbat M, Madhav T, et al. Left ventricular thrombus enhancement after intravenous injection of echogenic immunoliposomes: Studies in a new experimental model. Circulation 2002;105:2772–8
- Deshpande N, Needles A, Willmann JK. Molecular ultrasound imaging: Current status and future directions. Clin Radiol 2010;65:567–81
- Korpanty G, Carbon JG, Grayburn PA, Fleming JB, Brekken RA. Monitoring response to anticancer therapy by targeting microbubbles to tumor vasculature. Clin Cancer Res 2007;13:323–30
- Deshpande N, Ren Y, Foygel K, Rosenberg J, Willmann JK. Tumor angiogenic marker expression levels during tumor growth: Longitudinal assessment with molecularly targeted microbubbles and US imaging. Radiology 2011;258:804–11
- Ellegala DB, Leong-Poi H, Carpenter JE, Klibanov AL, Kaul S, Shaffrey ME, et al. Imaging tumor angiogenesis with contrast ultrasound and microbubbles targeted to alpha(v)beta3. Circulation 2003;108:336–41
- Barua A, Yellapa A, Bahr JM, Machado SA, Bitterman P, Basu S, et al. Enhancement of ovarian tumor detection with αvβ3 integrin-targeted ultrasound molecular imaging agent in laying hens: A preclinical model of spontaneous ovarian cancer. Int J Gynecol Cancer 2014;24:19–28
- Kuliszewski MA, Fujii H, Liao C, Smith AH, Xie A, Lindner JR, et al. Molecular imaging of endothelial progenitor cell engraftment using contrast-enhanced ultrasound and targeted microbubbles. Cardiovasc Res 2009;83:653–62
- Bachawal SV, Jensen KC, Lutz AM, Gambhir SS, Tranquart F, Tian L, et al. Earlier detection of breast cancer with ultrasound molecular imaging in a transgenic mouse model. Cancer Res 2013;73:1689–98
- Grouls C, Hatting M, Rix A, Pochon S, Lederle W, Tardy I, et al. Liver dysplasia: US molecular imaging with targeted contrast agent enables early assessment. Radiology 2013;267:487–95
- Khanicheh E, Mitterhuber M, Xu L, Haeuselmann SP, Kuster GM, Kaufmann BA. Noninvasive ultrasound molecular imaging of the effect of statins on endothelial inflammatory phenotype in early atherosclerosis. PLoS One 2013;8:e58761
- Khanicheh E, Qi Y, Xie A, Mitterhuber M, Xu L, Mochizuki M, et al. Molecular imaging reveals rapid reduction of endothelial activation in early atherosclerosis with apocynin independent of antioxidative properties. Arterioscler Thromb Vasc Biol 2013;33:2187–92
- Pysz MA, Foygel K, Rosenberg J, Gambhir SS, Schneider M, Willmann JK. Antiangiogenic cancer therapy: Monitoring with molecular US and a clinically translatable contrast agent (BR55). Radiology 2010;256:519–27
- Baron Toaldo M, Salvatore V, Marinelli S, Palama C, Milazzo M, Croci L, et al. Use of VEGFR-2 targeted ultrasound contrast agent for the early evaluation of response to sorafenib in a mouse model of hepatocellular carcinoma. Mol Imaging Biol 2014; in press
- Korpanty G, Carbon JG, Grayburn PA, Fleming JB, Brekken RA. Monitoring response to anticancer therapy by targeting microbubbles to tumor vasculature. Clin Cancer Res 2007;13:323–30
- Palmowski M, Huppert J, Ladewig G, Hauff P, Reinhardt M, Mueller MM, et al. Molecular profiling of angiogenesis with targeted ultrasound imaging: Early assessment of antiangiogenic therapy effects. Mol Cancer Ther 2008;7:101–9
- Sirsi SR, Flexman ML, Vlachos F, Huang J, Hernandez SL, Kim HK, et al. Contrast ultrasound imaging for identification of early responder tumor models to anti-angiogenic therapy. Ultrasound Med Biol 2012;38:1019–29
- Streeter JE, Herrera-Loeza SG, Neel NF, Yeh JJ, Dayton PA. A comparative evaluation of ultrasound molecular imaging, perfusion imaging, and volume measurements in evaluating response to therapy in patient-derived xenografts. Technol Cancer Res Treat 2013;12:311–21
- Flisikowska T, Kind A, Schnieke A. The new pig on the block: Modelling cancer in pigs. Transgenic Res 2013;22:673–80
- Kim H, Britton GL, Peng T, Holland CK, McPherson DD, Huang SL. Nitric oxide-loaded echogenic liposomes for treatment of vasospasm following subarachnoid hemorrhage. Int J Nanomedicine 2014;9:155–65
- Chadderdon SM, Belcik JT, Bader L, Kirigiti MA, Peters DM, Kievit P, et al. Proinflammatory endothelial activation detected by molecular imaging in obese nonhuman primates coincides with onset of insulin resistance and progressively increases with duration of insulin resistance. Circulation 2014;129:471–8
- Davidson BP, Chadderdon SM, Belcik JT, Gupta S, Lindner JR. Ischemic memory imaging in nonhuman primates with echocardiographic molecular imaging of selectin expression. J Am Soc Echocardiogr 2014;27:786–93
- Streeter JE, Gessner RC, Tsuruta J, Feingold S, Dayton PA. Assessment of molecular imaging of angiogenesis with three-dimensional ultrasonography. Mol Imaging 2011;10:460–8
- Streeter JE, Dayton PA. An in vivo evaluation of the effect of repeated administration and clearance of targeted contrast agents on molecular imaging signal enhancement. Theranostics 2013;3:93–8
- Bzyl J, Palmowski M, Rix A, Arns S, Hyvelin JM, Pochon S, et al. The high angiogenic activity in very early breast cancer enables reliable imaging with VEGFR2-targeted microbubbles (BR55). Eur Radiol 2013;23:468–75
- Hu X, Caskey CF, Mahakian LM, Kruse DE, Beegle JR, Decleves AE, et al. In vivo validation and 3D visualization of broadband ultrasound molecular imaging. Am J Nucl Med Mol Imaging 2013;3:336–49
- Lentacker I, De Cock I, Deckers R, De Smedt SC, Moonen CT. Understanding ultrasound induced sonoporation: Definitions and underlying mechanisms. Adv Drug Deliv Rev 2014;72:49–64
- Bao S, Thrall BD, Miller DL. Transfection of a reporter plasmid into cultured cells by sonoporation in vitro. Ultrasound Med Biol 1997;23:953–9
- McLaughlan J, Ingram N, Smith PR, Harput S, Coletta PL, Evans S, et al. Increasing the sonoporation efficiency of targeted polydisperse microbubble populations using chirp excitation. IEEE Trans Ultrason Ferroelectr Freq Control 2013;60:2511–20
- Skachkov I, Ying L, van der Steen AFW, de Jong N, Kooiman K. Targeted microbubble mediated sonoporation of endothelial cells in vivo. IEEE Trans Ultrason Ferroelectr Freq Control 2014;61:1661–7
- Phillips LC, Klibanov AL, Wamhoff BR, Hossack JA. Intravascular ultrasound detection and delivery of molecularly targeted microbubbles for gene delivery. IEEE Trans Ultrason Ferroelectr Freq Control 2012;59:1596–601
- Chang S, Guo J, Sun J, Zhu S, Yan Y, Zhu Y, et al. Targeted microbubbles for ultrasound mediated gene transfection and apoptosis induction in ovarian cancer cells. Ultrason Sonochem 2013;20:171–9
- Xie A, Belcik T, Qi Y, Morgan TK, Champaneri SA, Taylor S, et al. Ultrasound-mediated vascular gene transfection by cavitation of endothelial-targeted cationic microbubbles. JACC Cardiovasc Imaging 2012;5:1253–62
- Tlaxca JL, Rychak JJ, Ernst PB, Konkalmatt PR, Shevchenko TI, Pizzaro TT, et al. Ultrasound-based molecular imaging and specific gene delivery to mesenteric vasculature by endothelial adhesion molecule targeted microbubbles in a mouse model of Crohn's disease. J Control Release 2013;165:216–25
- Liu H, Chang S, Sun J, Zhu S, Pu C, Zhu Y, et al. Ultrasound-mediated destruction of LHRHa-targeted and paclitaxel-loaded lipid microbubbles induces proliferation inhibition and apoptosis in ovarian cancer cells. Mol Pharm 2014;11:40–8
- Yan F, Li X, Jin Q, Jiang C, Zhang Z, Ling T, et al. Therapeutic ultrasonic microbubbles carrying paclitaxel and LyP-1 peptide: Preparation, characterization and application to ultrasound-assisted chemotherapy in breast cancer cells. Ultrasound Med Biol 2011;37:768–79
- Pu C, Chang S, Sun J, Zhu S, Liu H, Zhu Y, et al. Ultrasound-mediated destruction of LHRHa-targeted and paclitaxel-loaded lipid microbubbles for the treatment of intraperitoneal ovarian cancer xenografts. Mol Pharm 2014;11:49–58
- Fan CH, Ting CY, Liu HL, Huang CY, Hsieh HY, Yen TC, et al. Antiangiogenic-targeting drug-loaded microbubbles combined with focused ultrasound for glioma treatment. Biomaterials 2013;34:2142–55
- McLaughlan J, Ingram N, Smith P, Harput S, Coletta P, Evans S, et al. Increasing the sonoporation efficiency of targeted polydisperse microbubble populations using chirp excitation. IEEE Trans Ultrason Ferroelectr Freq Control 2013;60:2511–20
- Fan Z, Kumon RE, Park J, Deng CX. Intracellular delivery and calcium transients generated in sonoporation facilitated by microbubbles. J Control Release 2010;142:31–9
- van Wamel A, Kooiman K, Harteveld M, Emmer M, ten Cate FJ, Versluis M, et al. Vibrating microbubbles poking individual cells: Drug transfer into cells via sonoporation. J Control Release 2006;112:149–55
- Kotopoulis S, Postema M. Microfoam formation in a capillary. Ultrasonics 2010;50:260–8
- Hu X, Kheirolomoom A, Mahakian LM, Beegle JR, Kruse DE, Lam KS, et al. Insonation of targeted microbubbles produces regions of reduced blood flow within tumor vasculature. Invest Radiol 2012;47:398–405
- Wood AK, Schultz SM, Lee WM, Bunte RM, Sehgal CM. Antivascular ultrasound therapy extends survival of mice with implanted melanomas. Ultrasound Med Biol 2010;36:853–7
- Rask-Andersen M, Zhang J, Fabbro D, Schioth HB. Advances in kinase targeting: Current clinical use and clinical trials. Trends Pharmacol Sci 2014;35:604–20
- Joo Ha H, Crum LA. Current status of clinical high-intensity focused ultrasound. IEEE EMBC 2009 Annu Int Conf; 2009 September 3–6; Minneapolis, MN, USA. pp 130–3
- Xiaoping L, Leizhen Z. Advances of high intensity focused ultrasound (HIFU) for pancreatic cancer. Int J Hyperthermia 2013;29:678–82
- Zhou Y, Wang Z, Chen Y, Shen H, Luo Z, Li A, et al. Microbubbles from gas-generating perfluorohexane nanoemulsions for targeted temperature-sensitive ultrasonography and synergistic HIFU ablation of tumors. Adv Mater 2013;25:4123–30