Abstract
Introduction. We have studied the functions of truncated apoE4 forms in vitro and in vivo in order to identify the domains of apoE4 required for the biogenesis of apoE-containing high-density lipoprotein (HDL). Results. We have found that apoE4-185, -202, -229, or -259 could promote ATP-binding cassette transporter A1 (ABCA1)-dependent cholesterol efflux in vitro, although less efficiently than Full-length apoE4, and had diminished capacity to activate lecithin cholesterol acyltransferase (LCAT). Formation of HDL in vivo was assessed by various methods following gene transfer in apolipoprotein A-I−/− × apoE−/− mice. Fast protein liquid chromatography of plasma showed that the truncated apoE forms, except apoE4-185, generated an apoE-containing HDL peak. Two-dimensional gel electrophoresis of plasma and electron microscopy showed that truncated apoE forms generated distinct HDL subpopulations and formed discoidal HDL particles which could be converted to spherical by co-administration of truncated apoE4-202 and LCAT. Conclusion. Overall, the in-vivo and in-vitro data are consistent and indicate that apoE4-185 is the shortest truncated form that supports formation of discoidal apoE4-containing HDL particles.
Abbreviations | ||
EM | = | electron microscopy |
apoE4 | = | apolipoprotein E4 |
Kmapp | = | apparent Km |
Vmaxapp | = | apparent Vmax |
ABCA1 | = | ATP-binding cassette transporter A1 |
HDL | = | high-density lipoprotein |
LCAT | = | lecithin cholesterol acyltransferase |
2D-GE | = | two-dimensional gel electrophoresis |
Key messages
Gene transfer in double-deficient apoA-I−/− × apoE−/− mice established that truncated apoE4 forms can generate discrete HDL subpopulations and discoidal HDL particles that can be converted to spherical by excess lecithin cholesterol acyltransferase (LCAT).
Consistent with the in-vivo data, in-vitro studies established that truncated apoE4 forms can promote ABCA1-dependent cholesterol efflux, which is the first step in HDL biogenesis, but activate LCAT poorly.
The ability of truncated apoE4 variants to form HDL differentiates apoE4 from apoA-I where the C-terminal domain is required for ABCA1-mediated cholesterol efflux and for the biogenesis of HDL.
Introduction
Apolipoprotein E (apoE) is a very important protein of the lipoprotein transport system, is essential for lipid homeostasis in the circulation, and is protective against atherosclerosis (Citation1–3).
Lipoprotein-bound apoE is the ligand for the low-density lipoprotein (LDL) receptor as well as other receptors in vitro (Citation4–6). In-vivo and in-vitro studies have shown that mutations in apoE that diminish binding of apoE-containing lipoproteins to the LDL receptor are associated with high plasma cholesterol levels and cause premature atherosclerosis in humans and experimental animals (Citation1–3). Recently, we have shown that apoE participates in the biogenesis of distinct apoE-containing high-density lipoprotein (HDL) species with the participation of ATP-binding cassette transporter A1 (ABCA1) and lecithin cholesterol acyltransferase (LCAT) (Citation7). The pathways of biogenesis of apoE- and apoA-I-containing HDL are similar (Citation7,Citation8). The combined properties of apoE to promote formation of apoE-containing HDL and to clear lipoprotein remnants explain, at least partially, the more pronounced contribution of apoE to atheroprotection as compared to apoA-I (Citation1,Citation9,Citation10). ApoE has three common isoforms (apoE2, apoE3, and apoE4) in humans (Citation11). In addition to its role in either the pathogenesis of or protection from cardiovascular disease, the apoE4 isoform is a risk factor for late-onset Alzheimer's disease (Citation12,Citation13). ApoE is the only medium-size apolipoprotein expressed in the brain (Citation14), and existing literature suggests that formation of apoE-containing HDL may have beneficial effects in the brain (Citation14–17).
The domains of apoE required for the biogenesis of apoE-containing HDL are not known. We have chosen to work with apoE4 due to the unique involvement of this isoform with both lipid homeostasis and with Alzheimer's disease (Citation12,Citation13). In this manuscript, we show that truncated apoE4 forms can promote ABCA1-dependent cholesterol efflux but have diminished capacity to activate LCAT in vitro. When expressed in double-deficient apoA-I−/− × apoE−/− mice, the truncated apoE forms generate mostly discoidal HDL particles that can be converted to spherical by excess LCAT and form discrete HDL subpopulations. The ability of the truncated apoE4 forms to promote cellular efflux of cholesterol in vitro is consistent with their ability to direct in vivo the formation of mostly discoidal HDL particles. This property of the truncated apoE4 variants differentiates apoE from apoA-I, where the C-terminal domain is required for the biogenesis of HDL particles. These newly identified functions of apoE are important for understanding the role of this protein, both in cardiovascular disease and in Alzheimer's disease.
Materials and Methods
Materials
Materials not mentioned in Methods have been obtained from sources described previously (Citation18,Citation19).
Methods
Recombinant adenoviruses construction. The recombinant adenoviruses were constructed as described using the Ad-Easy-1 system where the adenovirus construct is generated in BJ-5183 bacterial cells (Citation20,Citation21). Following large-scale infection of HEK293 cell cultures, the recombinant adenoviruses were purified by two consecutive CsCl2 ultracentrifugation steps, dialyzed, and titrated. Usually, titers of approximately 2–5 × 1010 pfu/mL were obtained.
Animal studies and RNA isolation and apoE mRNA quantitation. Male and female apoE−/− × apoA-I−/− double-deficient mice 8–10 weeks old were used in these studies. Groups of four or more mice were injected intravenously through the tail vein with a dose of 0.5–4 × 109 pfu of adenoviruses expressing truncated apoE forms. Blood was obtained from the tail vein after a 4-h fast preceding adenoviral injection and 1, 2, 3, and 4 days post infection. The plasma was isolated and stored at 4°C and -20°C. Four or more animals from each group were sacrificed 4 days post infection, hepatic RNA was isolated by the trizol method (Invitrogen), and the apoE mRNA levels were determined by quantitative PCR using the standard TaqMan thermal cycling program and Applied Biosystems probe sets for human apoE and 18s rRNA (Cat. #HJ00171168-M1 and 4319413E, respectively).
Fast protein liquid chromatography analysis of plasma (FPLC), lipid and apoE determinations. 12 μL of plasma were diluted 1:4 with PBS, and loaded onto a Superose 6 column in a SMART micro FPLC system (Pharmacia) and eluted with PBS. A total of 25 fractions of 50 μL volume each were collected for further analysis. Triglycerides and free cholesterol of plasma and the FPLC fractions were determined spectrophotometrically at 540 nm and 490 nm, respectively, as described previously (Citation20), using the plasma triglyceride kit (Sigma) and free cholesterol kit (Wako), according to the manufacturer's instructions. The total cholesterol (TC) in plasma was determined by hydrolysis of cholesterol esters and subsequent determination of the free cholesterol levels using cholesterol E kit (Wako). Cholesterol ester (CE) was determined by subtraction of free from total cholesterol levels. Plasma human apoE concentrations were measured using sandwich ELISA (Citation20).
Statistical analysis. Data are presented as the mean ± standard deviation. Statistically significant differences between two groups of mice were determined using Student's t test with unequal variance.
Density gradient ultracentrifugation and electron microscopy (EM) analysis. To assess the ability of full- length and truncated apoE forms to associate with different lipoproteins, an aliquot of 0.3 mL of plasma was fractionated by KBr density gradient ultracentrifugation, and the fractions were analyzed by SDS-PAGE as described previously (Citation22–24). Aliquots of the 5–8 fractions obtained by density gradient ultracentrifugation that contain most of the apoE were analyzed by EM. The photomicrographs were taken at × 75,000 magnification and enlarged three times as described previously (Citation25).
Non-denaturing two-dimensional electrophoresis. The distribution of HDL subfractions in plasma was analyzed by two-dimensional gel electrophoresis as described (Citation26). ApoE was detected by probing the membrane with a goat polyclonal anti-human apoE antibody (Meridian Biosciences K74190G; Saco, ME).
LCAT activation by full-length and truncated apoE4 forms. LCAT, apoA-I, full-length and truncated, and apoE4 forms were purified from the culture medium of HTB-13 cells infected with adenoviruses expressing the corresponding proteins as described previously (Citation27–31,Citation33). The 14C-cholesterol-labeled reconstituted HDL (rHDL) particles containing the various apoE4 forms used as substrate were prepared by the sodium cholate dialysis method (Citation31,Citation32). The enzymatic reactions carried out at different concentrations of apoE-containing rHDL were determined and the apparent kinetic parameters (Vmaxapp and Kmapp) were obtained by fitting the data to the Michaelis–Menten equation, using the Prism software (GraphPad Software, Inc.) as described (Citation22).
Cholesterol efflux studies. Efflux experiments were performed in HEK293 EBNA-T cells transfected with an ABCA1-expressing plasmid or a control plasmid (mock) as described (Citation18,Citation24), using 1 to 6 μM full-length apoA-I, apoE2, apoE3, apoE4 or truncated apoE4 forms as cholesterol acceptors at 37°C for 4 h. Efflux of [3H]cholesterol from the cells was expressed as the percentage of the radioactivity released in the medium relative to the total radioactivity in cells and medium. The net ABCA1- mediated efflux was determined by subtracting the cholesterol efflux of the cells transfected with the control plasmid (mock) from the cholesterol efflux of the cells transfected with the ABCA1 plasmid.
Results
Ability of apoE forms to promote ABCA1-mediated cholesterol efflux and to activate LCAT
The full-length apoA-I and apoE4 as well as the apoE4 variants were produced by large-scale cultures of HTB-13 cells in roller bottles following infection with adenoviruses expressing the corresponding protein and purified.
ABCA1-mediated cholesterol efflux represents the first step in the biogenesis of HDL. We have compared the ability of full-length apoA-I and apoE, as well as the ability of truncated apoE4 forms, to promote cholesterol efflux from HEK293 EBNA-T cells transfected with an ABCA1-expressing plasmid. It was found that all three natural apoE isoforms, apoE2, apoE3, and apoE4, at either 1 or 3 μM concentrations, have similar ability to promote ABCA1-dependent cholesterol efflux as compared to full-length apoA-I. On the other hand, the truncated apoE4 forms showed a concentration-dependent ability to promote cholesterol efflux. At 1 μM concentration, the cholesterol efflux for the shorter forms (apoE4-229, apoE4-202, apoE4-185) ranged from 17% to 30% of the full-length apoE4. At 3 μM concentration, the cholesterol efflux of E4-259 was 73% as compared to the full-length apoE4. At concentration of 6 μM, the efflux promoted by apoE4-185, -202, and -229 was 70%, 69%, and 86% of the full-length apoE4, respectively (). The ability of truncated apoE4 forms to promote ABCA1-dependent cholesterol efflux suggested that they may be able to participate in the biogenesis of HDL.
Figure 1. Functional properties of full-length and truncated apoE or apoA-I forms. A: Cholesterol efflux by full-length apoE and apoA-I and truncated apoE4 forms from HEK293 EBNA-T cells. The concentration of the acceptor apoE or apoA-I in the culture medium was either 1 or 3 or 6 μM, as indicated. Experiments were performed as described in Methods. Values are the mean ± standard deviation from two independent experiments performed in duplicate. B: Activation of LCAT by rHDL containing full-length and truncated apoE4 forms. Experiments were performed as described in Methods. A range of apoE concentrations were used in order to obtain the apparent kinetic parameters Kmapp and Vmaxapp and the catalytic efficiency Vmaxapp/Kmapp of the enzymatic reaction for each apoE form incorporated into POPC/cholesterol/apoE proteoliposomes. Values are the means ± standard deviation from three independent experiments performed in duplicate.
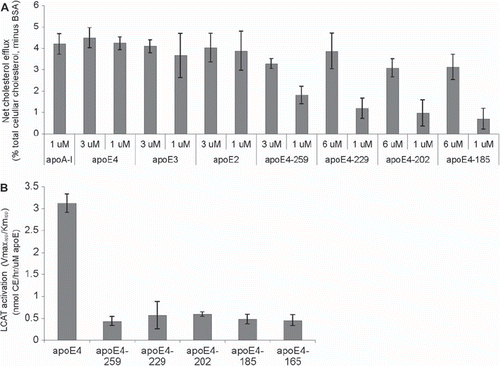
LCAT-mediated esterification of free cholesterol is required for the conversion of discoidal HDL to spherical HDL particles in vitro and in vivo. We have performed in-vitro LCAT activation assays using b-oleoyl-g-palmitoyl-L-a-phosphatidylcholine/ cholesterol/apoE (POPC/C/apoE) (rHDL) particles containing full-length or truncated apoE4 forms. Using different concentrations of apoE, we obtained the kinetic constants apparent Vmax (Vmaxapp) and apparent Km (Kmapp) for different apoE4 forms. This analysis showed that the catalytic efficiency (Vmaxapp/Kmapp) of the enzymatic reaction for truncated apoE4 forms was 14%–18% that of full-length apoE4 ().
Plasma lipid and apoE levels of apoA-I−/− × apoE−/− mice expressing full-length and truncated apoE4 forms
To map the domains of apoE4 required for the biogenesis of HDL, we have used adenovirus-mediated gene transfer of full-length and truncated apoE4 forms into apoA-I−/− × apoE−/− mice. These mice were chosen in order to rule out formation of apoE-containing HDL particles by the endogenous mouse apoE.
This analysis showed that when mice were infected with high doses of recombinant adenovirus (1.5 × 109 to 4 × 109 pfu), all truncated apoE4 forms cleared cholesterol without induction of hypertriglyceridemia ( and ). Similar clearance of cholesterol with induction of mild hypertriglyceridemia was observed when the mice were infected with low doses (5 × 108 pfu) of an adenovirus expressing full-length apoE4 ( and ).
Figure 2. Measurements following adenovirus-mediated gene transfer of full-length and truncated apoE forms in apoA-I−/− × apoE−/− mice. A: Plasma cholesterol, B: plasma triglycerides, C: hepatic apoE mRNA levels of apoA-I−/− × apoE−/− mice infected with recombinant adenoviruses expressing truncated and full-length apoE4 forms, and D: plasma apoE. Doses of adenovirus used were 4 × 109 pfu apoE4-185, 2 × 109 pfu apoE4-202, 1.5 × 109 pfu apoE4-229, 3 × 109 pfu apoE4-259, and 5 × 108 pfu full-length apoE4. Plasma lipids were determined on days 0 through 4, and hepatic apoE mRNA and plasma apoE levels were determined on day 4.
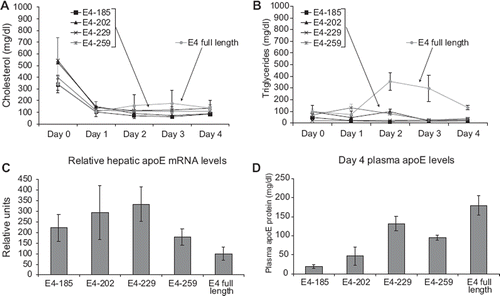
The dose of adenoviruses used was selected so that mice expressing the truncated apoE4 forms had comparable or higher hepatic apoE4 mRNA levels to the mRNA levels of mice expressing low doses of full-length apoE4 (). The plasma apoE levels 4 days post infection were higher in mice expressing full-length apoE4, were similar in mice expressing E4-229 and -259, and were reduced in mice expressing E4-202 or E4-185 as compared to mice expressing E4-229 or E4-259 ().
Assessment of the formation of HDL particles by FPLC, density gradient ultracentrifugation, electron microscopy, and two-dimensional gel electrophoresis
FPLC analysis of plasma showed that expression of all the truncated apoE4 forms tested, with the exception of apoE4-185, resulted in the formation of an HDL peak that was enriched in free cholesterol ( and ; Supplementary Figure 1A–D). Similarly enriched in free cholesterol were the peaks of VLDL, IDL, and LDL. Analysis of mice infected with low doses of adenoviruses expressing full-length apoE4 showed that the HDL peak consisted mostly of esterified cholesterol (CE/TC = 0.61) (). FPLC analysis of plasma of uninfected apoA-I−/− × apoE−/− mice showed the presence of a large VLDL peak with CE/TC ratio of 0.52 (Supplementary Figure 1D).
Figure 3. Fractionation of plasma by FPLC and density gradient ultracentrifugation. A, B: FPLC profiles of plasma from apoA-I−/− × apoE−/− mice infected with 1.5 × 109 apoE4-229 (A), 5 × 108 full-length apoE4 (B). The positions of VLDL/IDL and HDL fractions as well as the cholesterol ester to total cholesterol (CE/TC) ratio are indicated. The distribution of apoE in different lipoprotein fractions, as determined by Western blotting, is indicated below panels A and B. C, D: Distribution of apoE in different lipoprotein fractions following density gradient ultracentrifugation of plasma. Samples obtained from apoA-I−/− × apoE−/− mice 4 days post infection with adenovirus expressing apoE4-229 or full-length apoE4 were fractionated by density gradient ultracentrifugation and analyzed by SDS-PAGE as described in Methods. The density of each fraction and the position of apoE in the VLDL/IDL, LDL, and HDL fractions are indicated in each panel. Panel C shows analysis of plasma of mice infected with 1.5 × 109 apoE4-229. Similar distribution was obtained by analysis of plasma of mice infected with 2 × 109 pfu of apoE4-202, 3 × 109 apoE4-259 (see Supplementary Figure 2A–D). Panel D shows analysis of plasma of mice infected with 5 × 108 full-length apoE4.
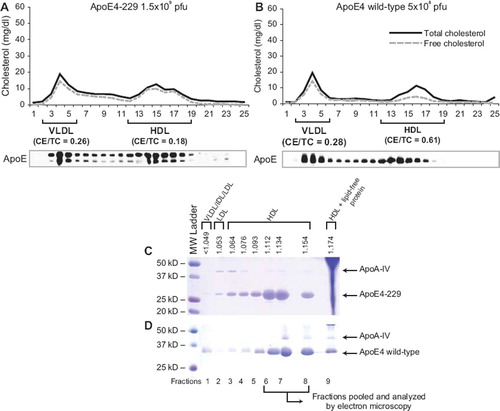
Density gradient ultracentrifugation of plasma of uninfected and infected apoA-I−/− × apoE−/− mice showed that apoE4-185 floats in the VLDL, IDL, LDL, and HDL2, and exists in low concentrations in plasma, whereas apoE4-202, -229, -259, and full-length apoE float predominantly in the HDL3 region, to a much lesser extent in the VLDL, IDL, LDL, and HDL2 region, and their concentration is much greater than that of apoE4-185 ( and ; Supplementary Figure 2A–D).
Fractions 6 to 8, which are enriched in apoE4 and float in the HDL density region, were analyzed further by electron microscopy. HDL obtained from mice expressing apoE4-185 contained a small number of discoidal particles (). HDL obtained from mice expressing E4-202 and -229 contained a large number of discoidal particles ( and ). HDL obtained from mice expressing E4-259 contained a mixture of discoidal and spherical particles (). HDL obtained from mice infected with low doses (5 × 108 pfu) of adenoviruses expressing full-length apoE4 contained only spherical particles (), whereas HDL obtained from mice infected with high doses (1 × 109 pfu) of adenoviruses expressing full-length apoE4 contained a mixture of spherical and discoidal particles (). HDL fractions 6 to 8 obtained from plasma of uninfected apoA-I−/− × apoE−/− or ABCA1−/− mice contained a sparse population of particles ().
Figure 4. Electron micrographs of HDL fractions separated by density gradient ultracentrifugation. Fractions of Figure 3C and D and Supplementary Figure 2A and D were pooled and analyzed by EM. The samples analyzed in panels A through I were obtained from mice expressing apoE4-185 (A), apoE4-202 (B), apoE4-229 (C), apoE4-259 (D), a low dose of apoE4 full-length (5 × 108 pfu) (E), high dose (1 × 109 pfu) apoE4 full-length (F), and uninfected apoA-I−/− × apoE−/− mice (G). A similar pattern was obtained in ABCA1−/− mice. (H) mice infected with 1.5 × 109 apoE4-202 pfu and 5 × 108 GFP. (I) HDL obtained from mice infected with 1.5 × 109 pfu apoE4-202 and 5 × 108 LCAT.
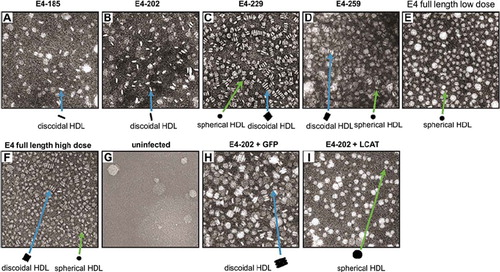
LCAT can promote the maturation of the discoidal HDL generated by the truncated form apoE4-202
To assess the ability of LCAT to convert discoidal HDL particles containing apoE4-202 into spherical particles, mice were co-infected with 1.5 × 109 pfu of adenovirus expressing apoE4-202 and 5 × 108 pfu adenovirus expressing human LCAT, or 1.5 × 109 pfu adenovirus expressing apoE4-202 and 5 × 108 pfu of adenovirus expressing GFP. Both treatments cleared cholesterol without induction of hypertriglyceridemia (Supplementary Figure 3A and B). Plasma apoE levels were reduced significantly in mice co-infected with adenoviruses expressing apoE4-202 and LCAT as compared to mice co- infected with adenoviruses expressing apoE4-202 and GFP (Supplementary Figure 3C and D), whereas the hepatic mRNA levels of the two groups were comparable (Supplementary Figure 3G).
FPLC analysis of plasma showed that the HDL cholesterol of mice treated with apoE4-202 and GFP was not esterified (CE/TC ratio = 0.04) whereas HDL cholesterol of mice treated with apoE4-202 and LCAT was esterified (CE/TC ratio = 0.65) (Supplementary Figure 3E and F).
Electron microscopy of the HDL fractions 6 and 7, corresponding to the fractions shown in Supplementary Figure 3C and D, showed that apoA-I−/− × apoE−/− mice infected with adenovirus expressing apoE4-202 and GFP form discoidal particles (), whereas the mice infected with apoE4-202 and LCAT formed mostly spherical particles (). These findings indicate that the discoidal apoE4-202-containing HDL particles can be converted to spherical by excess LCAT.
The formation of apoE4-containing HDL was also assessed by two-dimensional gel electrophoresis (2D-GE) that employs agarose gel electrophoresis in the first dimension and native PAGE in the second dimension. This analysis showed the formation of a small diffuse population of particles that were barely detectable in mice infected with high doses (4 × 109 pfu) of an adenovirus expressing apoE4-185 (). Infection of mice with adenoviruses expressing apoE4-202 and apoE4-229 promoted the formation of two distinct subpopulations of apoE-containing particles ( and ).
Figure 5. Two-dimensional gel electrophoresis of plasma of apoA-I−/− × apoE−/− mice infected with adenoviruses expressing full-length and truncated apoE4 forms. Plasma was obtained from mice infected with the following doses of adenovirus: 4 × 109 pfu apoE4-185 (A), 2 × 109 apoE4-202 (B), 1.5 × 109 apoE4-229 (C), 3 × 109 apoE4-259 (D), 5 × 108 pfu full-length apoE4 (E), and 1 × 109 pfu full-length apoE4 (F).
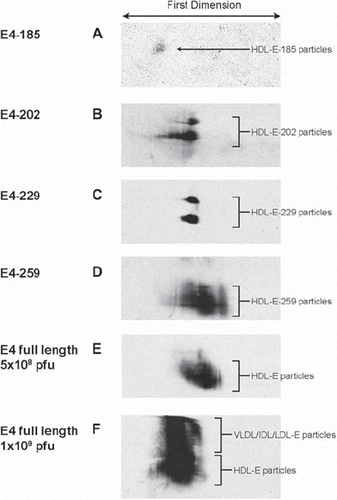
Infection of mice with adenoviruses expressing apoE4-259 produced a diffused population of apoE-containing particles (). A similar two-dimensional pattern was observed when mice were infected with low doses (5 × 108 pfu) of an apoE4-expressing adenovirus, which cleared plasma cholesterol and induced only mild hypertriglyceridemia (). However, when mice were infected with higher doses of an apoE4-expressing adenovirus (1 × 109 pfu), which increased plasma cholesterol and induced severe hypertriglyceridemia, an additional more slowly migrating population of lipoprotein particles was detected (). The more slowly migrating population most likely represents apoE-containing VLDL/IDL/LDL particles.
Discussion
Background
A series of recent studies have established that biogenesis of apoA-I-containing HDL is a continuous pathway where apoA-I and various participating proteins interact successively to form initially a lipidated intermediate and subsequently discoidal and spherical HDL particles (Citation34). Previous studies showed that C-terminal truncations that remove the 220 to 231 residues of apoA-I diminish the ability of the protein to promote cholesterol efflux and prevent formation of discoidal or spherical HDL particles (Citation18,Citation34,Citation35).
ApoE and apoA-I have striking structural and functional similarities as well as differences (Citation1,Citation18, Citation27,Citation35–40). ApoE contains N- and C-terminal domains which unfold independently (Citation36,Citation37), whereas apoA-I gives a single unfolding curve following chemical or thermal denaturation (Citation38). Both lipid-free apoA-I or apoE promote ABCA1-dependent cholesterol efflux which represents the first step in the biogenesis of HDL () (Citation18). In addition, similarly to apoA-I, lipid-bound apoE activates LCAT () (Citation41,Citation42) and promotes SR-BI-dependent cholesterol efflux (Citation23). Both apoA-I and apoE participate in the biogenesis of distinct HDL particles that contain the corresponding protein (Citation7,Citation34). The objective of this study was to assess the importance of the C-terminal region of apoE4 in the biogenesis of apoE-containing HDL.
A property of apoE that is not shared by apoA-I is its ability to bind to various cell receptors (Citation1–3) and promote in-vivo clearance of lipoprotein remnants and other apoE-containing lipoproteins via the LDL receptor (Citation1,Citation43). This may explain why apoE deficiency leads to severe atherosclerosis (Citation1), whereas apoA-I deficiency only exacerbates the atherosclerosis of susceptible mouse strains (Citation9,Citation10).
Truncated apoE4 forms promote the formation of discoidal HDL which can be converted to spherical by excess LCAT
The ability of truncated apoE forms to promote ABCA1-dependent cholesterol efflux at 3–6 μM concentration suggested that the product of ABCA1-apoE interactions might proceed further to form HDL in vivo. To address this question we employed adenovirus-mediated gene transfer of truncated apoE forms in apoA-I−/− × apoE−/− double-deficient mice.
The in-vivo experiment provided novel information on the ability of truncated apoE4 variants to form apoE4-containing HDL as well as the types of the HDL particles generated. Following infection with the recombinant adenoviruses, apoE4-185 was distributed in the VLDL/IDL/LDL region. However, all other truncated apoE4 forms were distributed predominantly in the HDL region. The build-up of apoE4 in the HDL or, in the case of apoE4-185, in the VLDL/IDL/LDL region inhibited the esterification of the cholesterol of HDL and VLDL/IDL/LDL. Based on this finding the expectation was that if HDL particles were formed they should have discoidal shape. Indeed, EM analysis established for the first time that apoE4-185 and -202 formed exclusively discoidal particles, and apoE4-229 and apoE4-259 formed a mixture of discoidal and spherical particles. The formation of discoidal HDL particles is consistent with the diminished capacity of the truncated apoE4 forms to activate LCAT in-vitro. A mixture of discoidal and spherical particles was also produced by high levels of expression of full-length apoE4 that induces dyslipidemia, whereas low levels of expression of apoE4 or apoE3 that clears the cholesterol of the double-deficient mice generated only spherical particles (; Supplementary Figure 4). The association between induction of dyslipidemia and formation of discoidal HDL particles was also observed in experiments with apoE−/− mice (Citation44).
The property of truncated apoE4 forms to promote formation of discoidal apoE-containing HDL differentiates apoE from apoA-I where deletion of the carboxy-terminal domain prevented the formation of discoidal or spherical HDL particles (Citation35). The esterification of the HDL cholesterol and the conversion of the discoidal to spherical HDL by excess LCAT suggest that the truncated apoE4 forms are poor activators of LCAT in vivo. It is also possible that mice that express the truncated apoE4 forms have LCAT insufficiency. This insufficiency may have resulted from a fast removal of complexes of LCAT with the minimally lipidated apoE forms or the discoidal HDL species containing truncated apoE forms by the kidney, as it happens for the discoidal apoA-I-containing HDL species (Citation45).
The distinct HDL subpopulations generated by apoE4-202 and apoE4-229 with similar mobility and size are very interesting. These HDL particles that have been observed for the first time are associated with the formation of discoidal-only HDL. In contrast, the formation of a more complex set of HDL species by apoE4-259 and low doses of full-length apoE4 may be indicative of formation of at least some mature spherical HDL particles.
Equally important is the observation that under conditions of dyslipidemia in addition to the apoE4-containing HDL particles we detect by two-dimensional gel electrophoresis larger-size lipoproteins which may represent apoE4-containing VLDL/IDL/LDL particles.
Overall the current study demonstrates that truncated apoE forms can generate apoE-containing discoidal HDL particles that can be converted to spherical by excess LCAT and that apoE4-185 is the shortest truncated apoE form that supports formation of discoidal apoE4-containing HDL particles.
Acknowledgements
We thank Konstantinos Drosatos, Andreas Kateifides, Gayle Forbes, and Adelina Duka for technical assistance and Anne Plunkett for preparing the manuscript.
Declaration of interest: This work was supported by grants from the National Institutes of Health (HL68216 and HL48739). A Vezeridis was supported by the NIH Predoctoral Training Grant (HL007969).
References
- Plump AS, Smith JD, Hayek T, Aalto-Setala K, Walsh A, Verstuyft JG, . Severe hypercholesterolemia and atherosclerosis in apolipoprotein E-deficient mice created by homologous recombination in ES cells. Cell. 1992;71:343–53.
- Schaefer EJ, Gregg RE, Ghiselli G, Forte TM, Ordovas JM, Zech LA, . Familial apolipoprotein E deficiency. J Clin Invest. 1986;78:1206–19.
- Reddick RL, Zhang SH, Maeda N. Atherosclerosis in mice lacking apo E. Evaluation of lesional development and progression. Arterioscler Thromb. 1994;14:141–7.
- Herz J, Willnow TE. Lipoprotein and receptor interactions in vivo. Curr Opin Lipidol. 1995;6:97–103.
- Kim DH, Iijima H, Goto K, Sakai J, Ishii H, Kim HJ, . Human apolipoprotein E receptor 2. A novel lipoprotein receptor of the low density lipoprotein receptor family predominantly expressed in brain. J Biol Chem. 1996; 271:8373–80.
- Takahashi S, Kawarabayasi Y, Nakai T, Sakai J, Yamamoto T. Rabbit very low density lipoprotein receptor: a low density lipoprotein receptor-like protein with distinct ligand specificity. Proc Natl Acad Sci U S A. 1992;89:9252–6.
- Kypreos KE, Zannis VI. Pathway of biogenesis of apolipoprotein E-containing HDL in vivo with the participation of ABCA1 and LCAT. Biochem J. 2007;403:359–67.
- Zannis VI, Koukos G, Drosatos K, Vezeridis A, Zanni EE, Kypreos KE, . Discrete roles of apoA-I and apoE in the biogenesis of HDL species: lessons learned from gene transfer studies in different mouse models. Ann Med. 2008;40 Suppl 1:14–28.
- Hughes SD, Verstuyft J, Rubin EM. HDL deficiency in genetically engineered mice requires elevated LDL to accelerate atherogenesis. Arterioscler Thromb Vasc Biol. 1997;17: 1725–9.
- Voyiaziakis E, Goldberg IJ, Plump AS, Rubin EM, Breslow JL, Huang LS. ApoA-I deficiency causes both hypertriglyceridemia and increased atherosclerosis in human apoB transgenic mice. J Lipid Res. 1998;39:313–21.
- Zannis VI, Just PW, Breslow JL. Human apolipoprotein E isoprotein subclasses are genetically determined. Am J Hum Genet. 1981;33:11–24.
- Zannis VI, Zanni EE, Makrides SC, Kardassis D, Aleshkov S. Role of apolipoprotein E in Alzheimer's disease. In: Catravas JD. NATO ASI Series, Life Sciences. New York: Plenum Press; 1998. p. 179–209.
- Corder EH, Saunders AM, Strittmatter WJ, Schmechel DE, Gaskell PC, Small GW, . Gene dose of apolipoprotein E type 4 allele and the risk of Alzheimer's disease in late onset families. Science. 1993;261:921–3.
- Boyles JK, Pitas RE, Wilson E, Mahley RW, Taylor JM. Apolipoprotein E associated with astrocytic glia of the central nervous system and with nonmyelinating glia of the peripheral nervous system. J Clin Invest. 1985;76:1501–13.
- Koldamova R, Staufenbiel M, Lefterov I. Lack of ABCA1 considerably decreases brain ApoE level and increases amyloid deposition in APP23 mice. J Biol Chem. 2005;280: 43224–35.
- Wahrle SE, Jiang H, Parsadanian M, Hartman RE, Bales KR, Paul SM, . Deletion of Abca1 increases Abeta deposition in the PDAPP transgenic mouse model of Alzheimer disease. J Biol Chem. 2005;280:43236–42.
- Wahrle SE, Jiang H, Parsadanian M, Kim J, Li A, Knoten A, . Overexpression of ABCA1 reduces amyloid deposition in the PDAPP mouse model of Alzheimer disease. J Clin Invest. 2008;118:671–82.
- Chroni A, Liu T, Gorshkova I, Kan HY, Uehara Y, von Eckardstein A, . The central helices of apoA-I can promote ATP-binding cassette transporter A1 (ABCA1)-mediated lipid efflux. Amino acid residues 220-231 of the wild-type apoA-I are required for lipid efflux in vitro and high density lipoprotein formation in vivo. J Biol Chem. 2003;278:6719–30.
- Liu T, Krieger M, Kan HY, Zannis VI. The effects of mutations in helices 4 and 6 of apoA-I on scavenger receptor class B type I (SR-BI)-mediated cholesterol efflux suggest that formation of a productive complex between reconstituted high density lipoprotein and SR-BI is required for efficient lipid transport. J Biol Chem. 2002;277:21576–84.
- Kypreos KE, Van Dijk KW, van Der Zee A, Havekes LM, Zannis VI. Domains of apolipoprotein E contributing to triglyceride and cholesterol homeostasis in vivo. Carboxyl- terminal region 203-299 promotes hepatic very low density lipoprotein-triglyceride secretion. J Biol Chem. 2001;276: 19778–86.
- He TC, Zhou S, da Costa LT, Yu J, Kinzler KW, Vogelstein B. A simplified system for generating recombinant adenoviruses. Proc Natl Acad Sci U S A. 1998;95:2509–14.
- Chroni A, Kan HY, Shkodrani A, Liu T, Zannis VI. Deletions of helices 2 and 3 of human apoA-I are associated with severe dyslipidemia following adenovirus-mediated gene transfer in apoA-I-deficient mice. Biochemistry. 2005;44: 4108–17.
- Chroni A, Nieland TJ, Kypreos KE, Krieger M, Zannis VI. SR-BI mediates cholesterol efflux via its interactions with lipid-bound ApoE. Structural mutations in SR-BI diminish cholesterol efflux. Biochemistry. 2005;44:13132–43.
- Chroni A, Duka A, Kan HY, Liu T, Zannis VI. Point mutations in apolipoprotein a-I mimic the phenotype observed in patients with classical lecithin:cholesterol acyltransferase deficiency. Biochemistry. 2005;44:14353–66.
- Li X, Kypreos K, Zanni EE, Zannis V. Domains of apoE required for binding to apoE receptor 2 and to phospholipids: Implications for the functions of apoE in the brain. Biochemistry. 2003;42:10406–17.
- Fielding CJ, Fielding PE. Two-dimensional nondenaturing electrophoresis of lipoproteins: applications to high-density lipoprotein speciation. Methods Enzymol. 1996;263:251–9.
- Chroni A, Kan HY, Kypreos KE, Gorshkova IN, Shkodrani A, Zannis VI. Substitutions of glutamate 110 and 111 in the middle helix 4 of human apolipoprotein A-I (apoA-I) by alanine affect the structure and in vitro functions of apoA-I and induce severe hypertriglyceridemia in apoA-I-deficient mice. Biochemistry. 2004;43:10442–57.
- Hill JS, O K, Wang X, Paranjape S, Dimitrijevich D, Lacko AG, . Expression and characterization of recombinant human lecithin:cholesterol acyltransferase. J Lipid Res. 1993;34:1245–51.
- Jin L, Lee YP, Jonas A. Biochemical and biophysical characterization of human recombinant lecithin: cholesterol acyltransferase. J Lipid Res. 1997;38:1085–93.
- Amar MJA, Shamburek RD, Foger B, Hoyt RF, Wood DO, Santamarina-Fojo S, . Adenovirus-mediated expression of LCAT in non-human primates leads to an antiatherogenic lipoprotein profile with increased HDL and decreased LDL. Circulation. 1998;98:35.
- Laccotripe M, Makrides SC, Jonas A, Zannis VI. The carboxyl-terminal hydrophobic residues of apolipoprotein A-I affect its rate of phospholipid binding and its association with high density lipoprotein. J Biol Chem. 1997;272:17511–22.
- Matz CE, Jonas A. Micellar complexes of human apolipoprotein A-I with phosphatidylcholines and cholesterol prepared from cholate-lipid dispersions. J Biol Chem. 1982;257: 4535–40.
- Li X, Kan HY, Lavrentiadou S, Krieger M, Zannis V. Reconstituted discoidal apoE-phospholipid particles are ligands for the scavenger receptor BI. The amino-terminal 1-165 domain of apoE suffices for receptor binding. J Biol Chem. 2002;277: 21149–57.
- Zannis VI, Chroni A, Krieger M. Role of apoA-I, ABCA1, LCAT, and SR-BI in the biogenesis of HDL. J Mol Med. 2006;84:276–94.
- Chroni A, Koukos G, Duka A, Zannis VI. The carboxy- terminal region of apoA-I is required for the ABCA1-dependent formation of alpha-HDL but not prebeta-HDL particles in vivo. Biochemistry. 2007;46:5697–708.
- Aggerbeck LP, Wetterau JR, Weisgraber KH, Wu CS, Lindgren FT. Human apolipoprotein E3 in aqueous solution. II. Properties of the amino- and carboxyl-terminal domains. J Biol Chem. 1988;263:6249–58.
- Wetterau JR, Aggerbeck LP, Rall SC Jr, Weisgraber KH. Human apolipoprotein E3 in aqueous solution. I. Evidence for two structural domains. J Biol Chem. 1988;263: 6240–8.
- Gorshkova IN, Liu T, Kan HY, Chroni A, Zannis VI, Atkinson D. Structure and stability of apolipoprotein a-I in solution and in discoidal high-density lipoprotein probed by double charge ablation and deletion mutation. Biochemistry. 2006;45:1242–54.
- Nolte RT, Atkinson D. Conformational analysis of apolipoprotein A-I and E-3 based on primary sequence and circular dichroism. Biophys J. 1992;63:1221–39.
- Wilson C, Wardell MR, Weisgraber KH, Mahley RW, Agard DA. Three-dimensional structure of the LDL receptor- binding domain of human apolipoprotein E. Science. 1991;252:1817–22.
- Steinmetz A, Kaffarnik H, Utermann G. Activation of phosphatidylcholine-sterol acyltransferase by human apolipoprotein E isoforms. Eur J Biochem. 1985;152:747–51.
- Chen CH, Albers JJ. Activation of lecithin: cholesterol acyltransferase by apolipoproteins E-2, E-3, and A-IV isolated from human plasma. Biochim Biophys Acta. 1985;836: 279–85.
- Kypreos KE, Zannis VI. LDL receptor deficiency or apoE mutations prevent remnant clearance and induce hypertriglyceridemia in mice. J Lipid Res. 2006;47:521–9.
- Kypreos KE, Van Dijk KW, Havekes LM, Zannis VI. Generation of a recombinant apolipoprotein E variant with improved biological functions: hydrophobic residues (LEU-261, TRP-264, PHE-265, LEU-268, VAL-269) of apoE can account for the apoE-induced hypertriglyceridemia. J Biol Chem. 2005;280:6276–84.
- Koukos G, Chroni A, Duka A, Kardassis D, Zannis VI. LCAT can rescue the abnormal phenotype produced by the natural ApoA-I mutations (Leu141Arg)Pisa and (Leu159Arg) FIN. Biochemistry. 2007;46:10713–21.