Abstract
Mixed protein-surfactant micelles are used for in vitro studies and 3D crystallization when solutions of pure, monodisperse integral membrane proteins are required. However, many membrane proteins undergo inactivation when transferred from the biomembrane into micelles of conventional surfactants with alkyl chains as hydrophobic moieties. Here we describe the development of surfactants with rigid, saturated or aromatic hydrocarbon groups as hydrophobic parts. Their stabilizing properties are demonstrated with three different integral membrane proteins. The temperature at which 50% of the binding sites for specific ligands are lost is used as a measure of stability and dodecyl-β-D-maltoside (‘C12-b-M’) as a reference for conventional surfactants. One surfactant increased the stability of two different G protein-coupled receptors and the human Patched protein receptor by approximately 10°C compared to C12-b-M. Another surfactant yielded the highest stabilization of the human Patched protein receptor compared to C12-b-M (13°C) but was inferior for the G protein-coupled receptors. In addition, one of the surfactants was successfully used to stabilize and crystallize the cytochrome b6 f complex from Chlamydomonas reinhardtii. The structure was solved to the same resolution as previously reported in C12-b-M.
Introduction
Mixed protein-surfactant micelles are often used for in vitro studies when solutions of pure, monodisperse integral membrane proteins are needed (Garavito and Ferguson-Miller Citation2001). Because integral membrane proteins evolved in a lipid bilayer environment, the exchange of lipid for surfactant frequently causes a destabilization of the protein. This can result in modified activity followed by increased rate of denaturation, increased susceptibility to protease attack, accelerated heat denaturation, oxidative damage, loss of activity regulation and lack of crystallization (Tanford and Reynolds Citation1976, Tate Citation2010).
At the beginning of membrane protein research only surfactants developed as detergents for consumer or industrial use were available, and many of them were inhomogenous. In vitro studies of solubilized membrane proteins and structural work (Michel and Oesterhelt Citation1980, Rosenbusch et al. Citation1981) led to an increasing demand in tailored surfactants. In the micellar solution the protein should be functional and stable for extended periods of time and the micellar belt attached to the protein should be small in order to leave much of the polar protein surfaces uncovered for crystal lattice contacts (Michel Citation1983).
Studies of the micellar mass revealed the relation between the chemical structure of a surfactant and the size of its micelle. It was found to depend upon the ratio of the polar cross-section and the length of the extended hydrophobic chain (Israelachvili et al. Citation1976). In the case of spherical micelles, the latter matches the radius of the hydrophobic core. With alkyl chains as hydrophobic moieties of surfactants proteins require a length between an extended octyl and a dodecyl chain (Iwata Citation2003).
Small micelles are formed by β-octylglucoside and lauryldimethylamineoxide (LDAO) with masses of 26 kDa (He et al. Citation2000) and 16 kDa (Timmins et al. Citation1988), respectively. Medium-sized micelles are formed by dodecyl-β-maltoside with a mass of 66 kDa (Timmins et al. Citation1988).
Attention has recently turned to the eukaryotic membrane proteins which are often far less stable than their prokaryotic counterparts. Some of these had been studied very early because of important function and sufficient abundance in natural sources. One example is bovine rhodopsin, a G protein-coupled receptor (GPCR). The Meta I–Meta II equilibrium is a sensitive monitor for a native-like occupation of conformations. In rod disk membranes the equilibrium of dark-adapted bovine rhodopsin is on the Meta I side while in most surfactants it is shifted towards Meta II (Schleicher et al. Citation1987). Only one surfactant, the steroid-based digitonin, retained the native equilibrium (Szundi et al. Citation2005). Baldwin and Hubbell (Citation1985) suggested that shifts in the Meta I–Meta II equilibrium of rhodopsin in artificial lipid bilayers result from changes in the interfacial tension or excess surface free energy (ESFE). This indicated that surfactant micelles that maintain native-like conditions (henceforward referred to as ‘mild conditions’) require a certain surface tension at the interface between the hydrophobic moiety of the surfactant and the transmembrane surface of the protein. The interactions between the hydrophobic surface of the membrane protein and the hydrocarbon groups of lipids or surfactants will be dominated by van der Waals contacts. Consequently it was assumed (Schleicher et al. Citation1987), that the ratio of the densities of van der Waals contacts which the hydrophobic moiety of a lipid or surfactant experiences in the bulk of the micelle and at the surface with the protein, respectively, determines its ESFE. For example, ratios near one lead to a low value and to a destabilizing surfactant while higher ratios lead to a high value and a mild surfactant.
It was further assumed that this ratio differs for a protein in a lipid bilayer and in a micelle assuming that both contain alkyl chains as hydrophobic moieties. In a bilayer in liquid crystalline phase, the alkyl chains assemble by more-or-less parallel packing with a restricted set of conformations distributed around the all-trans conformation (Senak et al. Citation1991), allowing for a high number of van der Waals contacts in the bulk of the bilayer. Alkyl chains adjacent to the irregularly shaped membrane-embedded protein surface will thus feature an ESFE because they experience a smaller density of van der Waals contacts than chains in the bulk and/or because they adopt a specific subset of conformations complementary to the protein surface, which raises their entropy. According to Marsh and Horvath (Citation1998), the second possibility is unlikely.
In a micelle, however, the alkyl chains must adopt a broad distribution of conformations to pack into a spheroidal or ellipsoidal aggregate (Szleifer and Ben-Shaul Citation1985, Holler and Callis Citation1989). The number of van der Waals contacts in the bulk will thus be smaller and the difference in density of van der Waals contacts of bulk alkyl chains compared to interface alkyl chains will also be smaller. The surface tension experienced by the protein thus will be lower than in the bilayer. A way to increase the ESFE in a micelle therefore should be to use surfactants with less flexible hydrophobic moieties, so that their ability to form van der Waals contacts in the bulk is increased and with the protein surface decreased. This could explain the ‘mildness’ of digitonin. To test this concept, a compound was synthesized, which contained a hydrophobic allyloxybiphenyl group esterified with an octaoxyethylene headgroup (Schleicher et al. Citation1987). It was expected that the rigid phenyl ring systems should increase the ESFE but still possess sufficient flexibility to form smaller micelles than digitonin. The results supported the hypothesis.
However, the solubilizing efficiency of the biphenyl compound was low and it did not retain the functionality of all proteins tested. Therefore, in the work described here we synthesized a series of surfactants by exchanging one or both aromatic cycles by cyclohexyl groups.
The allyloxybiphenyl group was changed to an alkoxyphenylcyclohexyl group or an alkylbicyclohexyl group. The alkyl was either a butyl or a propyl group. The polar headgroup was changed to a maltosyl because of stabilising effects (Xie and Timasheff Citation1997) and because of its compactness compared to ethyleneoxide groups.
These maltoside surfactants were made in pure anomeric forms because of known differences in solubility and surfactant action between anomers (Brown et al. Citation1970, Focher et al. Citation1989, Hunte et al. Citation2005). Finally, to exclude oxygen from the hydrophobic moiety which was suspected to render the hydrophobic part more aggressive due to its electronegativity, we synthesized propylphenylcyclohexylmaltoside and its butyl variant.
Methods
The Supplementary information available online includes detailed descriptions of the following:
Synthesis of the novel surfactants PoPC-a-M, BoPC-a-M, PPC-b-M, BPC-b-M, PCC-a-M, BCC-a-M;
Determination of micellar mass by SANS and SAXS;
Temperature stability of turkey β1-adrenergic receptor β1AR34-324;
Expression, purification and temperature stability of hSmo and hPtc;
Purification and structure analysis of cytochrome b6f;
Thermostability of β1AR34-324
In brief, the truncated wild-type turkey β1 receptor (β1AR34-324) was functionally expressed in Escherichia coli with a maltose binding protein fused to the N-terminus and a His-tag at the C-terminus. β1AR34-324 was solubilized and purified from membranes with C12-b-M. Aliquots were used to exchange the detergent for PCC-a-M, BPC-b-M, PPC-b-M and C12-b-M. The solubilized protein was incubated at the specified temperature for 30 min, quenching the reaction on ice, and 3H-dihydroalprenolol (3H-DHA) added to determine the amount of binding by the receptor. Receptor-bound and free radioligand were separated by gel filtration. The temperature was determined at which half of the binding was lost (‘T50’).
Thermostability of hSmo and hPtc
In brief, hSmo and hPtc carrying a multitag affinity purification sequence (MAP) at their C-terminal part were solubilized from membrane fractions expressing hSmo-MAP or hPtc-MAP with C12-b-M and loaded on a calmodulin column. Surfactant exchange was achieved by washing with buffers supplemented with either PCC-a-M, PPC-b-M, BPC-b-M or C12-b-M. SPR sensorgrams were measured using a sensor chip with a ligand-coated flow cell, CPN for Smo or N-SHh for Ptc, and a control, unliganded flow cell as described (Joubert et al. Citation2009, Nehmé et al. Citation2010). Prior to measurement, receptors were incubated for 30 min at temperatures ranging from 4–70°C. The percentage of remaining activity with respect to an unheated control was determined. The inflection point of the sensorgram signals, which was assumed to be equivalent to T50, was determined by fitting with a Boltzmann sigmoidal equation.
Crystal structure of cytochrome b6 f complex
In brief, cytochrome b 6 f was solubilized and purified in C12-b-M. The surfactant was exchanged for 0.2 mM PCC-a-M by Ni-NTA chromatography. All subsequent steps including crystallization were performed in 0.2 mM PCC-a-M. Crystals isomorphic to those in C12-b-M were obtained. Diffraction data to 2.8 Å were collected at the synchrotron SOLEIL (St Aubin, France). A model containing 8 PCC-a-M molecules was built and refined. Plastoquinol plasto cyanine oxidoreductase activity was measured as described (Pierre et al. Citation1995).
Results
Structures of the surfactants and their physicochemical data
Members of three surfactant families were synthesized:
4-(4′-alkoxyphenyl)-trans-cyclohexyl-α-d-maltoside family : Propoxy (‘PoPC-a-M’) and Butoxy (‘BoPC-a-M’);
trans-4-(4′-alkylphenyl)cyclohexyl-β-d-maltoside family: Propyl (‘PPC-b-M’) and Butyl (‘BPC-b-M’);
trans-4-(trans-4′-alkylcyclohexyl)cyclohexyl-α-d-maltoside family: Propyl (‘PCC-a-M’) and Butyl (‘BCC-a-M’).
Structures are shown in . Critical micelle concentrations are given in .
Figure 1. Representatives of the three surfactant families with rigid ring systems tested in this work: 4-(4′-propoxyphenyl)-trans-cyclohexyl-α-D-maltoside (PoPC-a-M), (1); trans-4-(4′-propylphenyl)cyclohexyl-β-D-maltoside (PPC-b-M), (2); trans-4-(trans-4′-propylcyclohexyl)cyclohexyl-α-D-maltoside (PCC-a-M), (3).
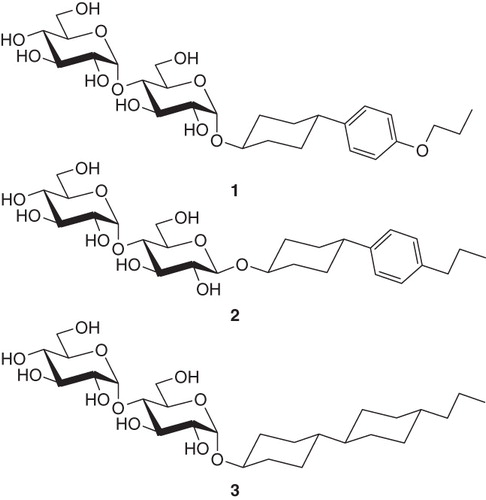
Table I. Physicochemical data of new surfactants and of C12-b-M.
Physicochemical data
Assuming a fully extended all-trans conformation, the radius of the hydrophobic core of micelles from the widely-used n-dodecyl-β-D-maltoside (C12-b-M) would be 13.7 Å (1.25 Å per C-C bond). For PCC-a-M, using 7.46 Å for the length of an all-trans bicyclohexyl group (Walz et al. Citation1989) and 3.76 Å for an all-trans propyl moiety, a maximal radius of 11.2 Å results, which resembles the length of an extended decyl chain. With regard to the critical micelle concentration (cmc), C12-b-M (0.17 mM) is more polar than PCC-a-M (0.036 mM) as expected from the larger number of hydrocarbon groups in the hydrophobic moiety of the latter.
The exchange of an aromatic group for a cyclohexyl group in PoPC-a-M and PPC-b-M increases the cmc to 0.41 mM and 1.18 mM, respectively, reflecting the relative polarity of an unsaturated as compared to a saturated six-membered hydrocarbon ring. The cmc-s decrease slightly between room temperature and 50°C (see ).
The micellar mass was determined by small-angle neutron scattering (SANS) for PoPC-a-M and BCC-a-M and by Small angle X ray scattering (SAXS) for PCC-a-M. The micellar radius was determined by SANS for PopC-a-M and BCC-a-M and by dynamic light scattering for PPC-b-M and BPC-b-M ( and Supplementary information, available online). The surfactants C12-b-M and PCC-a-M form micelles of similar mass (66 kDa (Timmins et al. Citation1988) and 63 kDa, respectively). BCC-a-M forms larger micelles (85 kDa), while those of PoPC-a-M are smaller (36 kDa). The cone-shaped phenyl-cyclohexyl group of PoPC-a-M likely facilitates packing into a smaller micelle whereas the brick-shaped bicyclohexyl group promotes a micelle of smaller curvature.
Thermostability of a β1-adrenergic receptor in the surfactants
The surfactants were tested on a truncated version of the turkey β1 adrenergic receptor (β1AR34-324), a GPCR, which also contains a C-terminal His6 tag (Warne et al. Citation2003, Citation2009, Serrano-Vega et al. Citation2008). Functional β1AR34-324 was expressed in E. coli. The receptors were solubilized with C12-b-M, bound to NiNTA beads and a chromatographic procedure was performed to exchange C12-b-M for PCC-a-M, BPC-b-M, PPC-b-M as described in Supplementary information, available online. A T50 of 40°C for β1AR34-324 in PCC-a-M indicated that the surfactant stabilized the receptor compared to C12-b-M, for which a T50 of 30°C was observed ( and ). In contrast, β1AR34-324 in either PPC-b-M or BPC-b-M was less stable than in C12-b-M, with apparent T50-s of 21°C and 25°C, respectively. Thus PCC-a-M significantly stabilizes β1AR34-324 in comparison to C12-b-M.
Figure 2. Thermostability of β1AR34-324 in mild surfactants. The receptor was partially purified in C12-b-M by Ni2+-affinity chromatography with the surfactant exchange performed in the final column washes. The receptor was eluted in the relevant surfactant and the thermostability was immediately assessed by heating for 30 min, quenching on ice and measuring the amount of functional receptor remaining using a 3H-DHA binding assay (Supplementary information, available online): red squares, PCC-a-M, T50 = 40°C; yellow triangles, C12-b-M, T50 = 30°C; green inverted triangles, BPC-b-M, T50 = 25°C; blue diamonds, PPC-b-M, T50 = 21°C. The results shown are from a single representative experiment performed in duplicate with error bars representing the SEM. Other experiments performed using the surfactants above, but not all simultaneously, gave similar apparent T50-s.
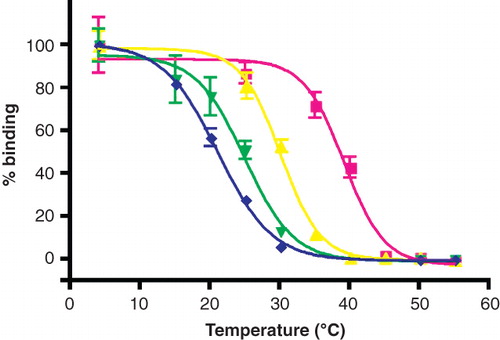
Table II. T50 values (°C).
Thermostability of Patched and Smoothened solubilized in the novel surfactants
The Sonic Hedgehog (SHh) signalling pathway involves two membrane receptors: Patched (Ptc), a 12-transmembrane domain protein receptor of the SHh protein, and Smoothened (Smo), a GPCR responsible for the transduction of the signal (Varjosalo and Taipale Citation2008). For in vitro studies, human Ptc and human Smo fused with a multi-affinity tag (MAP) at their C-terminus have been expressed at the plasma membrane of the yeast Saccharomyces cerevisiae and purified in C12-b-M in a native and stable conformation, as assessed by ligand binding using Surface Plasmon Resonance (SPR) studies (Joubert et al. Citation2009, Nehmé et al. Citation2010) (Supplementary information, available online).
C12-b-M was exchanged for each of the surfactants and as a control for C12-b-M, i.e., 2 mM each of C12-b-M, PCC-a-M, PPC-b-M and BPC-b-M. Western blots of 8% SDS PAGE gels loaded with hPtc and hSmo purified in the four surfactants and probed with anti-hemagglutinin (HA) antibodies directed against the HA tag of the MAP at their C-terminus showed bands at apparent molecular weights of approximately 180 and 90 kDa corresponding to hPtc and hSmo, respectively (Supplementary Figure S1), suggesting that the surfactants maintained both receptors in a soluble state similar as in C12-b-M.
The ability of purified hSmo and hPtc to bind their ligands was tested using SPR. Purified hSmo and hPtc were injected in both the reference flow cell (Fc1) and the CPN-(for hSmo) or Sonic Hedgehog-(for hPtc) coupled flow cell (Fc2). See Supplementary information, available online, for details. The difference between the sensorgrams recorded on Fc2 and Fc1 gave signals varying between 150 and 700 RU for the two proteins in each of the four surfactants, indicating that hSmo and hPtc were able to interact with their respective ligand after purification in each surfactant. The observed variations in the SPR response could be due to differences in the protein amount injected, but also due to other factors like variations in the refractive index of the surfactants.
For thermostability experiments, hSmo and hPtc samples were incubated for 30 min at increasing temperatures prior to SPR analysis as described above and under Supplementary information. The percentage of remaining native hSmo was determined by comparing the amount of binding (in RU) after heating with its unheated control. T50 was calculated as described in Materials and Methods and Supplementary information. hSmo () exhibited comparable thermostability in BPC-b-M and in C12-b-M (T50 = 39.8 ± 3°C, 39.4 ± 0.9°C respectively, ), and lower thermostability in PPC-b-M (T50 = 31 ± 2.7°C, ), whereas its thermostability was enhanced by about 12°C in PCC-a-M (T50 = 52.1 ± 0.7°C, ). These results indicate that PCC-a-M strongly stabilizes the GPCR hSmo in solution.
Figure 3. Thermostability of the human receptors of the Hedgehog pathway, hPtc and hSmo, in mild surfactants. Samples of hPtc or hSmo purified in C12-b-M, PCC-a-M, PPC-b-M or BPC-b-M were heated 30 min at increasing temperatures ranging from 4–70°C prior to injection on the reference flow cell (Fc1) and measure flow cell (Fc2) (coupled with N-SHh for hPtc and with CPN for hSmo experiments). Sensorgrams were recorded and the percentage of remaining native hSmo (a) and hPtc (b) was determined (SI). Red squares correspond to PCC-a-M; yellow triangles to C12-b-M; green inverted triangles to BPC-b-M and blue diamonds to PPC-b-M. The results shown are from a single representative experiment performed in triplicate with error bars representing the SEM. Several other experiments were performed and gave similar apparent T50-s.
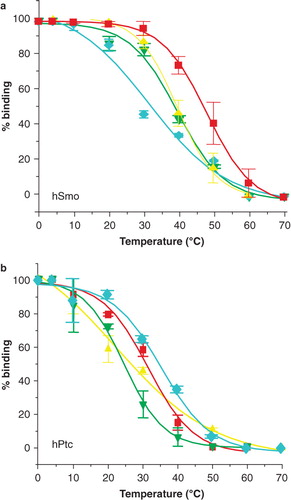
hPtc () showed an apparent T50 in C12-b-M of about 23°C (). It was enhanced by about 3°C, 9°C and 13°C in BPC-b-M (T50 = 26.4 ± 0.1°C), PCC-a-M (T50 = 32 ± 1.5°C) and PPC-b-M (T50 = 36.4 ± 1.7°C) respectively (). These results indicate that hPtc is more stable in these surfactants than in C12-b-M, PPC-b-M being the best surfactant for hPtc.
Enzymatic activity and structural analysis of cytochrome b6 f complex from Chlamydomonas reinhardtii
The cytochrome b6f complex was purified with C12-b-M, as described by Stroebel et al. (Citation2003), except that the detergent was exchanged for PCC-a-M in the last chromatographic step on NiNTA (Supplementary information, available online). This exchange of 0.2 mM C12-b-M for 0.2 mM PCC-a-M during the purification of the protein did not affect the biochemical properties of the cytochrome b6 f complex. The oxidoreductase activity of the complex (Pierre et al. Citation1995) and its stability over extended storage at 4°C were similar to those observed in 0.2 mM C12-b-M (), i.e., at a concentration slightly above the detergent's cmc. However, while the b 6 f complex inactivates rapidly in solutions of C12-b-M when the concentration is raised above the cmc (Breyton et al. Citation1997), it is only marginally affected in PCC-a-M even at 5 mM concentration ().
Figure 4. Stability of cytochrome b6 f exposed to low (0.2 mM) or high (5 mM) concentrations of either C12-b-M or PCC-a-M. (Top) Migration of the b6 f complex in sucrose gradients (10–30% w/w) prepared in 20 mM Tris/HCl buffer, pH 8.0, containing either 0.2 or 5 mM of either C12-b-M or PCC-a-M. Freshly prepared b6f (4.9 μM) in 0.2 mM C12-b-M [Stroebel et al. Citation2003] was layered on the top of the gradients and ultracentrifuged for 4 h at 4°C at 55,000 rpm (260,000 g) in a Beckman TLS 55 rotor. Monomerization, as observed in the presence of 5 mM C12-b-M, correlates with the inactivation of the complex (see references Breyton et al. Citation1997, Popot Citation2010). (Bottom) Kinetics of inactivation of the b6f complex in the presence of either 0.2 or 5 mM of either C12-b-M (circles, diamonds) or PCC-a-M (squares, triangles). The dimer bands were collected from the gradients and their electron-transfer activity assayed as described [Pierre et al. Citation1995] immediately after collection (t = 0) and after storage at 4°C in the dark for up to 10 days.
![Figure 4. Stability of cytochrome b6 f exposed to low (0.2 mM) or high (5 mM) concentrations of either C12-b-M or PCC-a-M. (Top) Migration of the b6 f complex in sucrose gradients (10–30% w/w) prepared in 20 mM Tris/HCl buffer, pH 8.0, containing either 0.2 or 5 mM of either C12-b-M or PCC-a-M. Freshly prepared b6f (4.9 μM) in 0.2 mM C12-b-M [Stroebel et al. Citation2003] was layered on the top of the gradients and ultracentrifuged for 4 h at 4°C at 55,000 rpm (260,000 g) in a Beckman TLS 55 rotor. Monomerization, as observed in the presence of 5 mM C12-b-M, correlates with the inactivation of the complex (see references Breyton et al. Citation1997, Popot Citation2010). (Bottom) Kinetics of inactivation of the b6f complex in the presence of either 0.2 or 5 mM of either C12-b-M (circles, diamonds) or PCC-a-M (squares, triangles). The dimer bands were collected from the gradients and their electron-transfer activity assayed as described [Pierre et al. Citation1995] immediately after collection (t = 0) and after storage at 4°C in the dark for up to 10 days.](/cms/asset/38b0bad7-a969-4905-8eeb-68758b154ac7/imbc_a_552440_f0004_b.jpg)
Crystallization in the presence of PCC-a-M occurred within a few days under similar conditions as in C12-b-M. The crystals obtained were isomorphous and with a diffraction quality similar to the best crystals obtained in C12-b-M. The structure was solved by difference Fourier techniques (see Supplementary information). As expected, the overall fold of the complex is almost identical to that in C12-b-M (Stroebel et al. Citation2003) (), albeit with a weaker electron density for the mobile domain of the Rieske protein. The latter is due to a lower occupancy of the Q0 site by the inhibitor tridecylstigmatellin which is probably not related to the use of PCC-a-M, but to the handling and batches of the inhibitor.
Figure 5. Crystal structure of the dimer of cytochrome b6f with the localisation of the structured PCC-a-M molecules (8 per monomer) seen from the membrane in CPK representation. The stromal side is at the top.
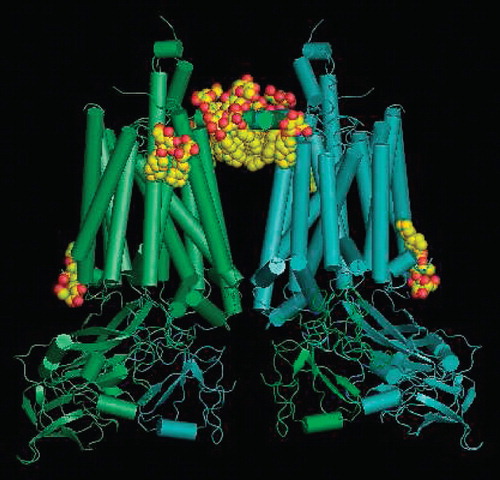
Major differences in the electron density maps obtained in the presence of PCC-a-M vs. C12-b-M are located at the protein-lipid interface. They were attributed to PCC-a-M molecules according to the following criteria: (1) Positive densities in the difference Fourier map between the two sets of data, and (2) thicker densities as expected for cyclohexyl groups, which are bulkier than the alkyl chains of lipids or of C12-b-M. While these criteria allowed the identification of eight PCC-a-M molecules, only two of them show well-defined densities for maltosyl groups. Seven PCC-a-M molecules are facing the stroma (Supplementary Figure S2a, available online) and only one the lumen (Supplementary Figure S2b, available online). On the stromal side, PCC-a-M molecules are located near or in the large cleft that lies between the two monomers. They can be divided into two sets: The first one contains five PCC-a-M molecules interacting mainly with the amphiphilic helix a (Zhang et al. Citation1998, Stroebel et al. Citation2003) of cytochrome b 6, which crosses the cleft and connects the two monomers. Two of these molecules have well-defined headgroups and substitute for two C12-b-M molecules. One of them exhibits only minor positional and conformational changes, while the other exhibits a drastic reorientation of its headgroup as compared to C12-b-M, putatively due to the α-glycosidic linkage. The three remaining molecules of this set have less well defined electron densities for the maltosyl group. In the second set, two molecules are further away from the cleft and interact with the transmembrane segment of the Rieske protein. The single PCC-a-M molecule visible on the luminal face of the membrane interacts with the C-terminal end of Subunit 4 and with a lipid molecule.
Discussion
Some of the surfactants stabilize membrane proteins against temperature-induced denaturation
For structural work on labile membrane proteins, improving thermostability has been shown to be advantageous (Warne et al. Citation2008). Detergent-solubilized membrane proteins are more thermolabile than their membrane-embedded forms. For the turkey β1-adrenergic receptor in C12-b-M, the apparent T50 is 30°C while in other surfactants like LDAO or β-octylglucoside, which are more suitable for crystallizing small membrane proteins, T50 values could not be determined, because the receptor was inactivated immediately upon solubilization (Warne et al. Citation2009). In membranes from birds or mammals, one would expect for labile proteins a minimal T50 of 40°C. The low T50 values observed in micellar solution thus are in accord with the general experience that many membrane proteins are destabilized upon detergent solubilization. One approach to solve this problem is to stabilize the membrane protein by suitable mutations. For example, in the turkey β1-adrenergic receptor, a combination of six point mutations increased the T50 by 21°C compared to the wild-type receptor, which allowed its crystallization and structure determination to 2.7 Å resolution (Warne et al. Citation2008).
Another approach, as adopted here, is to search for milder surfactants. We hypothesized that conformationally restricted groups in the hydrophobic part lead to mild surfactants. Combinations of cyclohexyl rings and aromatic rings were tried. The cone-like shape of a phenyl-cyclohexyl group as in PoPC-a-M, PPC-b-M and BPC-b-M was expected to increase the curvature and thereby to reduce the size of the micelles, while the brick-like shape of the cyclohexyl-cyclohexyl group was expected to act in the opposite way. This was indeed observed by SANS and SAXS (). Because conformationally restricted groups cannot be well-packed into a spheroidal micelle core, propyl or butyl groups were attached to the end of the hydrophobic moiety, resulting in an overall hydrophobic length comparable to that of C12-b-M.
In order to assess the properties of the surfactants and to compare them with the widely used detergent C12-b-M, thermostability measurements and crystallization experiments were undertaken. Two GPCRs, turkey β1-adrenergic receptor and hSmo, were both thermostabilized in PCC-a-M by about 12°C compared to C12-b-M (, and ). In PPC-b-M and BPC-b-M, however, the GPCRs show the same or lower T50 values than in C12-b-M.
However, the latter compounds stabilize the hPtc receptor (, ), with an apparent T50 of 36°C in PPC-b-M compared to 23°C in C12-b-M. This seems to indicate that aromatic π-electron systems stabilize some but destabilize other proteins. Destabilization is conceivable if there is an aromatic network in the transmembrane region (Haeger et al. Citation2010). Edge-to-face aromatic-aromatic interactions (Burley and Petsko Citation1986) could then affect the native structure of the protein. This weak electrostatic interaction of detergent molecules may in other cases be beneficial because it generates a lateral interaction between surfactant molecules which should contribute to the ESFE.
PCC-a-M achieves temperature stabilizations of the β1-adrenergic receptor by about 10°C, which is about half of the values reported for a six-residue mutant used for structure analysis (Serrano-Vega et al. Citation2008, Warne et al. Citation2008, Shibata et al. Citation2009). The design concept thus can be expected to lead to compounds which are instrumental for crystallizing labile membrane proteins.
PCC-a-M was therefore tested for crystallization of the b6 f complex from C. reinhardtii. This complex is quite unstable in conventional surfactants which induce dissociation of the dimeric complex associated with loss of the Rieske protein (Breyton et al. Citation1997). The structure explains this observation by the swapping of the soluble part of the flexible Rieske protein between the two monomers and by the presence of a large hydrophobic cleft separating the two monomers. This cleft is important for the catalytic mechanism of the complex since it permits plastoquinone exchange between the Qo and Qi sites located on opposite sides of the membrane. In situ, the cleft has to be filled with lipids of sufficient flexibility to avoid impairment of this exchange. The localization of several well-packed surfactant molecules in the cleft and near the transmembrane helix of the Rieske protein contributes to explaining how detergents may disrupt the complex, as observed with C12-b-M at concentrations above the cmc. To overcome this problem, several approaches have been used in the past: Working at concentrations near the cmc and/or addition of lipids (Pierre et al. Citation1995) to counterbalance the delipidating effect of detergent (Zhang et al. Citation2003). The mildness of PCC-a-M made it possible to work under similar conditions as with C12-b-M, but without the burden of an extremely high sensitivity to changes in surfactant concentration, a crucial issue for the crystallization of membrane proteins.
Packing differences between hydrophobic micellar and bilayer cores which are relevant to the design of the surfactants
According to our hypothesis, alkyl chains interact less strongly in micelles than in lipid bilayers. This is supported by experiments comparing enthalpies of alkyl chain methylene groups in micelles and bilayers. Heerklotz and Epand (Citation2001) compared enthalpies of melting of hydrocarbon chains in bulk oil and in lipid bilayers which had been determined by Phillips et al. (Citation1969). For the transition of a methylene group from a liquid crystalline lipid bilayer into a molten bulk hydrocarbon phase a ΔΔH value of approximately +2 kJ/mol per methylene group was found. This value suggests stronger mutual interactions in the bilayer as compared to bulk oil, putatively caused by partial alignment of the alkyl chains in the former.
In a micelle, the order of the hydrocarbon chains formed by an alkylic surfactant will differ from both of these (Dill and Flory Citation1981, Menger and Doll Citation1984) but should resemble more the bulk oil than the bilayer (Tanford Citation1974).
This supports our concept that hydrocarbons in an alkylic micelle core mediate a lower ESFE at the micelle-protein interface than a liquid crystalline bilayer and is in accord with ESFE estimations by Marsh (Citation2007).
Membrane proteins may also be adapted to the increasing order of methylene groups towards the polar headgroup in lipids (Seelig and Seelig Citation1974, Petrache et al. Citation2000), which is possibly opposite in micelles (Néry et al. Citation1986). Preferential rigidity of a surfactant in the apolar part adjacent to its polar headgroup thus could also contribute to mildness.
The concept to use rigid groups to enhance ESFE in other surfactants
The reduced chain order and increased enthalpy in micelles as compared to bilayers suggests that alkylic detergents have a lower ESFE than bilayer lipids. Rigid groups in the hydrophobic moiety of surfactants may lead to an increase in ESFE by a combination of improved lateral packing in the bulk of the micelle core and reduced ability to adopt conformations complementary to the protein or protein-lipid surface (Supplementary Figure S3, available online).
Another recently reported family of mild surfactants, hemifluorinated surfactants (HF), may be based on a similar principle. Perfluoroalkyl chains are both bulkier and more rigid than hydrocarbon chains so that gauche conformations are more restricted. Moreover they are more hydrophobic than alkyl chains and not miscible with the latter (Krafft and Riess Citation1998). This led to the design of HF surfactants (Breyton et al. Citation2004, Popot Citation2010) which is reminiscent of the design explored here. The cytochrome b6 f complex and hSmo show excellent temporal stability in some HF surfactants (Breyton et al. Citation2010, Nehmé et al. Citation2010).
Conclusions
Our work suggests that surfactants with an increased ESFE may help to retain functionality of labile eukaryotic integral membrane proteins in micellar solution. According to our hypothesis, rigid groups in the hydrophobic moieties should increase van der Waals interactions of surfactants in the bulk of the micelle and decrease interactions with the hydrophobic surface of membrane proteins, increasing the ESFE. In addition, weak interactions between the hydrophobic moieties of a surfactant as, for instance, aromatic edge-to-face interactions, can also be expected to increase ESFE.
The general ESFE concept proven of value here, offers many opportunities to devise mild surfactants which may be instrumental for in vitro functional, as well as crystallographic studies of labile membrane proteins.
Note added in proof
Recently we found that PCC-a-M can be used for solubilization of membranes and chromatographic purification of membrane proteins. We tried the β1AR34-324, two P-type ATPases, a secondary active transporter and a Cys-loop receptor. In all these cases it worked out.
Supplementary Figures S1–S3
Download MS Word (4.3 MB)Acknowledgements
Dr Eike Poetsch and Dr Alfred Blume helped at various stages of the work with stimulating discussions. We thank David Pauron and the UMR Interactions Biotiques et Santé Végétale (INRA/CNRS/Université de Nice Sophia Antipolis, France) for the access to the Biacore 3000, and Marielle Bauzan (Unité de fermentation IMM/IFR88 CNRS Marseille, France) for fermentor cultures. We are grateful to the synchrotron SOLEIL for providing beamtime and to Pierre Legrand for assistance in data collection on the beamline Proxima I. We also acknowledge the ESRF for provision of synchrotron radiation facilities and we would like to thank Petra Pernot and Adam Round for assistance in using beamline ID14-eh3. We gratefully acknowledge the donation of resynthesized PCC-a-M by Dr C. Kolar, Glycon GmbH, Luckenwalde, Germany, for late control experiments.
Declaration of interest: Most of us were supported by the European Community Specific Targeted Research Project grant “Innovative Tools for Membrane Protein Structural Proteomics” (STREP-IMPS, LSHG-CT-2005-513770). Part of this work was supported by the foundation France Cancer, the Conseil Général des Alpes Maritimes. The authors report no conflicts of interest. The authors alone are responsible for the content and writing of the paper.
References
- Baldwin PA, Hubbell WL. 1985. Effects of lipid environment on the light-induced conformational changes of rhodopsin. 1. Absence of metarhodopsin II production in dimyristoylphosphatidylcholine recombinant membranes. Biochemistry 24:2624–2632.
- Breyton C, Tribet C, Olive J, Dubacq JP, Popot JL. 1997. Dimer to monomer conversion of the cytochrome b6f complex. Causes and consequences. J Biol Chem 272:21892–21900.
- Breyton C, Chabaud E, Chaudier Y, Pucci B, Popot JL. 2004. Hemifluorinated surfactants: a non-dissociating environment for handlingmembrane proteins in aqueous solutions? FEBS Lett 564:312–318.
- Breyton C, Pucci B, Popot J-L. 2010. Amphipols and fluorinated surfactants: Two alternatives to surfactants for studying membrane proteins in vitro. In: Mus-Veteau I, editor. Heterologous expression of membrane proteins: Methods and protocols. Totowa, NJ: The Humana Press; Vol. 601. pp 219–245.
- Brown GM, Dubreuil FM, Ichhaporla FM, Desnoyers JE. 1970. Synthesis and properties of some a-D-alkyl glucosides and mannosides: Apparent molal volumes and solubilization of nitrobenzene in water at 25°C. Can J Chem 48:2525–2531.
- Burley SK, Petsko GA. 1986. Dimerization energetics of benzene and aromatic amino acid side chains. J Am Chem Soc 108:7995–8001.
- Dill KA, Flory PJ. 1981. Molecular organization in micelles and vesicles. Proc Natl Acad Sci USA 78:676–680.
- Focher B, Savelli G, Torri G, Vecchio G, McKenzie, DC, Nicoli DF, 1989. Micelles of 1-alkyl glucoside and maltoside: Anomeric effects on structure and induced chirality. Chem Phys Lett 158:491–494.
- Garavito RM, Ferguson-Miller S. 2001. Surfactants as tools in membrane biochemistry. J Biol Chem 276:32403–32406.
- Haeger S, Kuzmin D, Detro-Dassen S, Lang N, Kilb M, Tsetlin V, 2010. An intramembrane aromatic network determines pentameric assembly of Cys-loop receptors. Nat Struc Biol 70:90–99.
- He LZ, Garamus V, Niermeyer B, Helmholz H, Willumeit R. 2000. Determination of micelle structure of octyl-β-glucoside in aqueous solution by small angle neutron scattering and geometric analysis. J Mol Liquids 89:239–249.
- Heerklotz H, Epand RM. 2001. The enthalpy of acyl chain packing and the apparent water-accessible apolar surface area of phospholipids. Biophys J 80:271–279.
- Holler F, Callis JB. 1989. Conformation of the hydrocarbon chains of sodium dodecyl sulfate molecules in micelles: An FTIR study. J Phys Chem 93:2053–2058.
- Hunte C, Screpanti E, Venturi M, Rimon A, Padan E, Michel H, 2005. Structure of a Na1/H1 antiporter and insights into mechanism of action and regulation by pH. Nature 453:1197–1202.
- Israelachvili JN, Mitchell DJ, Ninham BW. 1976. Theory of self-assembly of hydrocarbon amphiphiles into micelles and bilayers. J Chem Soc Faraday Transact II 72:1525–1568.
- Iwata S. 2003. Methods and results in crystallization of membrane proteins. LaJolla, CA: International University Line.
- Joubert O, Nehmé R, Fleury D, De Rivoyre M, Bidet M, Polidori A, Ruat M, Pucci B, Mollat P, Mus-Veteau I. 2009. Functional studies of membrane-bound and purified human Hedgehog receptor Patched expressed in yeast. Biochim Biophys Acta 1788:1813–1821.
- Krafft MP, Riess JG. 1998. Highly fluorinated amphiphiles and colloidal systems, and their applications in the biomedical field. A contribution. Biochimie 80:489–514.
- Marsh D, Horvath LI. 1998. Structure, dynamics and composition of the lipid-protein interface. Perspectives from spin-labelling. Biochim Biophys Acta 1376:267–296.
- Marsh D. 2007. Lateral pressure profile, spontaneous curvature frustration, and the incorporation and conformation of proteins in membranes. Biophys J 93:3884–3899.
- Menger FM, Doll DWJ. 1984. On the structure of micelles. J Am Chem Soc 106:1109–1113.
- Michel H, Oesterhelt D. 1980. Three-dimensional crystals of membrane proteins: Bacteriorhodopsin. Proc Natl Acad Sci USA 77:1283–1285.
- Michel H. 1983. Crystallization of membrane proteins. Trends Biochem Sci 8:56–59.
- Nehmé R, Joubert O, Bidet M, Lacombe B, Polidori A, Pucci B, Mus-Veteau I. 2010. Stability study of the human G-protein coupled receptor, smoothened. Biochim Biophys Acta 1798:1100–1110.
- Néry H, Söderman O, Canet D, Walderhaug H, Lindman B. 1986. Surfactant dynamics in spherical and nonspherical micelles. A nuclear magnetic resonance study. J Phys Chem 90:5802–5808.
- Petrache HI, Dodd SW, Brown MF. 2000. Area per lipid and acyl length distributions in fluid phosphatidylcholines determined by 2H NMR spectroscopy. Biophys J 79:3172–3192.
- Phillips MC, Williams RM, Chapman D. 1969. On the nature of hydrocarbon chain motions in liquid crystals. Chem Phys Lip 3:234–244.
- Pierre Y, Breyton C, Kramer D, Popot JL. 1995. Purification and characterization of the cytochrome b6f complex from Chlamydomonas reinhardtii. J Biol Chem 270:29342–29349.
- Popot J-L. 2010. Amphipols, nanodiscs, and fluorinated surfactants: Three non-conventional approaches to studying membrane proteins in aqueous solutions. Ann Rev Biochem 79:737–775.
- Rosenbusch JP, Garavito RM, Dorset DL, Engel A. 1981. Structure and function of a pore-forming transmembrane protein: High resolution studies of a bacterial porin. In: Peeters H, editor. Protides of the biological fluids. Oxford: Pergamon Press. pp 171–174.
- Schleicher A, Hofmann KP, Finkelmann H, Welte W. 1987. Deoxylysolecithin and a new biphenyl surfactant as solubilizing agents for bovine rhodopsin. Functional test by formation of metarhodopsin II and binding of G-protein. Biochemistry 26:5908–5916.
- Seelig A, Seelig J. 1974. The dynamic structure of fatty acyl chains in a phospholipid bilayer measured by deuterium magnetic resonance. Biochemistry 13:4839–4845.
- Senak L, Davies MA, Mendelsohn R. 1991. A quantitative IR study of hydrocarbon chain conformation in alkanes and phospholipids: CH2 wagging modes in disordered bilayer and HII phases. J Phys Chem 95:2565–2571.
- Serrano-Vega MJ, Magnani F, Shibata S, Tate CG. 2008. Conformational thermostabilisation of the β-adrenergic receptor in a surfactant-resistant form. Proc Natl Acad Sci USA 105:877–882.
- Shibata Y, White JF, Serrano-Vega MJ, Magnani F, Aloia AL, Grisshammer R, 2009. Thermostabilization of the neurotensin receptor NTS1. J Mol Biol 390:262–277.
- Stroebel D, Choquet Y, Popot J-L, Picot D. 2003. An atypical heme in the cytochrome b6f complex. Nature 426:413–418.
- Szleifer I, Ben-Shaul A. 1985. Chain organization and thermodynamics in micelles and bilayers. II. Model calculations. J Chem Phys 83:3612–3620.
- Szundi I, Lewis JW, Kliger DS. 2005. Effect of digitonin on the rhodopsin meta I–meta II equilibrium. Photochem Photobiol 81:866–873.
- Tanford C. 1974. Theory of micelle formation in aqueous solutions. J Phys Chem 78:2469–2479.
- Tanford C, Reynolds JA. 1976. Characterization of membrane proteins in surfactant solutions. Biochim Biophy Acta 457:133–170.
- Tate CG. 2010. Practical considerations of membrane protein instability during purification and crystallization. In: Mus-Veteau I, editor. Heterologous expression of membrane proteins: Methods and protocols. Series: Methods in molecular biology. Totowa, NJ: Humana Press; Vol. 601. pp 187–203.
- Timmins PA, Leonhard M, Weltzien HU, Wacker T, Welte W. 1988. A physical characterization of some surfactants of potential use for membrane protein crystallization. FEBS Lett 238:361–368.
- Varjosalo M, Taipale J. 2008. Hedgehog: Functions and mechanisms. Genes Dev 22:2454–2472.
- Walz L, Haase W, Eidenschink R. 1989. The crystal and molecular structures of four homologous, mesogenic trans,trans-4,4′-dialkyl-(1α,1′-bicyclohexyl)-4ß-carbonitril (CCNs). Mol Cryst Liq Cryst 168:169–182.
- Warne T, Chirnside J, Schertler GF. 2003. Expression and purification of truncated, non-glycosylated turkey beta-adrenergic receptors for crystallization. Biochim Biophys Acta 1610:133–140.
- Warne T, Serrano-Vega MJ, Baker JG, Moukhametzianov R, Edwards PC, Henderson R, 2008. Structure of a β1-adrenergic G-protein coupled receptor. Nature 454:486–491.
- Warne T, Serrano-Vega MJ, Tate CG, Schertler GFX. 2009. Development and crystallization of a minimal thermostabilised G protein coupled receptor. Prot Exp Purif. 65:204–213.
- Xie G, Timasheff SN. 1997. The thermodynamic mechanism of protein stabilization by trehalose. Biophys Chem 64:25–43.
- Zhang Z, 1998. Electron transfer by domain movement in cytochrome bc1. Nature 392:677–684.
- Zhang H, Kurisu G, Smith JL, Cramer WA. 2003. A defined protein-surfactant-lipid complex for crystallization of integral membrane proteins: The cytochrome b6f complex of oxygenic photosynthesis. Proc Natl Acad Sci USA 100:5160–5163.