Abstract
Glycosylphosphatidylinositol (GPI)-anchored proteins have been regarded as typical cell surface proteins found in most eukaryotic cells from yeast to man. They are embedded in the outer plasma membrane leaflet via a carboxy-terminally linked complex glycolipid GPI structure. The amphiphilic nature of the GPI anchor, its compatibility with the function of the attached protein moiety and the capability of GPI-anchored proteins for spontaneous insertion into and transfer between artificial and cellular membranes initially suggested their potential for biotechnological applications. However, these expectations have been hardly fulfilled so far. Recent developments fuel novel hopes with regard to: (i) Automated online expression, extraction and purification of therapeutic proteins as GPI-anchored proteins based on their preferred accumulation in plasma membrane lipid rafts, (ii) multiplex custom-made protein chips based on GPI-anchored cell wall proteins in yeast, (iii) biomaterials and biosensors with films consisting of sets of distinct GPI-anchored binding-proteins or enzymes for sequential or combinatorial catalysis, and (iv) transport of therapeutic proteins across or into relevant tissue cells, e.g., enterocytes or adipocytes. Latter expectations are based on the demonstrated translocation of GPI-anchored proteins from plasma membrane lipid rafts to cytoplasmic lipid droplets and eventually further into microvesicles which upon release from donor cells transfer their GPI-anchored proteins to acceptor cells. The value of these technologies, which are all based on the interaction of GPI-anchored proteins with membranes and surfaces, for the engineering, production and targeted delivery of biomolecules for a huge variety of therapeutic and biotechnological purposes should become apparent in the near future.
Introduction
The cell membrane is a complex fluid mosaic composed of phospholipids, cholesterol and proteins (Singer and Nicolson Citation1972). The majority of membrane-bound proteins consists of extracellular and intracellular proteinaceous domains separated by hydrophobic transmembrane proteinaceous domains embedded in the lipid bilayer. However, proteins can also be anchored to cell membranes through the post-translational attachment of a covalently linked glycosylated form of phosphatidylinositol (Low Citation1989a, Englund Citation1993, Nosjean et al. Citation1997). These glycosylphosphatidylinositol (GPI) anchors are not only found in many different tissues of all mammals studied to date (Brewis et al. Citation1995), but also in yeast, protozoa (Ferguson Citation1999) and, recently, in algae and archaebacteria (Orlean and Menon Citation2007). The complete structure of a GPI anchor was first determined for the trypanosomal variant surface glycoprotein (VSG) (Ferguson et al. Citation1985), and rat brain Thy-1 (Homans et al. Citation1985). Although only few complete structures have been determined to date, a highly conserved core structure quickly appeared. In contrast, a unifying view about the physiological functions and pathophysiological roles of GPI-anchored proteins is far from being established 25 years later. Nevertheless, GPI-anchored proteins have attracted much interest in basic research during the past two decades. However, despite their unique biophysical and cell biological properties, such as amphiphilic nature, plasma membrane or cell wall localization, intra-, trans- and intercellular trafficking, significant biotechnological applications have not been realized so far, at least according to commonly availably data bases.
This review summarizes initial findings and claims about the potential biotechnological use of plasma membrane GPI-anchored proteins and combines them with very recent data on their intra- and transcellular targeting in as well as purification from mammalian cells. In consequence, novel concepts for the oral administration and cellular penetration of GPI-modified protein and nucleic acid drugs are currently emerging. Furthermore, examples for the advantageous use of cell wall GPI-anchored proteins in the construction of protein chips and novel biomaterials are presented.
Characteristics of GPI-anchored proteins
Structure
Transmembrane proteins span both the cytoplasmic and outer leaflets of the plasma membrane via a stretch of 15–25 hydrophobic amino acids and thereby expose protein domains of variable size both in the cytoplasm and at the cell surface. In contrast, GPI-anchored proteins are embedded in the outer leaflet of the plasma membrane, exclusively, via the covalently attached GPI anchor and thereby expose their protein moiety at the cell surface, only. The carboxy-terminus of a GPI-anchored protein is linked through a phosphoethanolamine bridge to the highly conserved glycan core, -6mannoseα1-2mannoseα1-6mannoseα1-4glucosamineα1-6myo-inositol- (Fujita and Kinoshita Citation2010), with the inositol residue representing the head group of the phospholipid component. The fatty acyl chains of the phosphatidylinositol moiety embed the GPI anchor in the cell membrane (Nosjean et al. Citation1997, Ferguson Citation1999).
The glycan core can be variously modified with side chains (Deeg et al. Citation1992, McConville and Ferguson Citation1993), such as fatty acyl (e.g., palmitate), phosphoethanolamine and sugar (e.g., mannose, galactose, sialic acid) groups. An additional fatty acid, such as palmitic acid, is often detected at the 2-hydroxyl position of the inositol ring, which renders the GPI anchor resistant to cleavage by phosphatiylinositol-specific phospholipase C (McConville and Ferguson Citation1993). The phosphoethanolamine side chain, attached to either the second or third mannose of the glycan core, is only found in higher eukaryotes, but not in protozoa (Ferguson Citation1999). The most common side chain attached to the first mannose residue is another mannose moiety. Complex side chains, such as the N-acetylgalactosamine-containing polysaccharides attached to the third mannose of the glycan core, are found in both mammalian and protozoan GPI structures. The core glucosamine is rarely modified. Depending on the protein and species of origin, the lipid portion of the phosphoinositol ring is a diacylglycerol, an alkylacylglycerol or a ceramide (McConville and Ferguson Citation1993). The fatty acyl moieties of the lipid portions vary in length, ranging from 14–28 carbons, and can be either saturated or unsaturated. During biosynthesis and even after attachment to the protein moiety the GPI anchor is structurally remodelled by exchange of their fatty acyl moieties in the course of specific transesterification reactions (Fujita and Kinoshita Citation2010). Taken together, the structural microheterogeneity of GPI anchors is due to: (i) The glycan core that can be branched by additional sugar and phosphoethanolamine residues, (ii) possible acylation of the inositol ring, and (iii) nature of the aliphatic chains of the phosphatidylinositol moiety.
Topology
GPI-anchored proteins seem to preferentially associate with lipid rafts, i.e., dynamic membrane microdomains (10–200 nm) enriched in glycolipids, sphingolipids, cholesterol, and certain types of lipidated proteins (Low Citation1989a, Milhiet et al. Citation2002, Munro Citation2003). Lipid rafts organize the plasma membrane into a series of discrete smaller domains that can serve as platforms for diverse cellular functions, such as vesicular trafficking and signal transduction (Brown and London Citation1998, Varma and Mayor Citation1998, Müller and Frick Citation1999, Rajendran and Simons Citation2005). Lipid rafts are hypothesized to form by the self-association of (glyco)sphingolipids, favored by their long and mostly saturated hydrocarbon chains that allow them to pack tightly in a phospholipid bilayer. Cholesterol molecules are believed to fill the voids between the neighbouring (glyco)sphingolipids. The presence of cholesterol is required for the function and formation of lipid rafts, as they are disrupted upon depletion of cellular cholesterol (Varma and Mayor Citation1998, Müller and Frick Citation1999, Munro Citation2003). Due to tight packing of the (glyco)sphingolipids, lipid rafts are believed to be less fluid than the surrounding phospholipid bilayer. The highly ordered environment of the lipid rafts may also allow for the close packing of GPI-anchored proteins.
From an experimental point of view, lipid rafts are typically characterized by their insolubility at 4°C in the non-ionic detergent Triton X-100. This has become the most widely used assay for the existence of lipid rafts (Brown Citation1992, Brown and Rose Citation1992, Rajendran and Simons Citation2005), although these detergent-resistant membranes might not represent the physiological status of lipid rafts. GPI-anchored proteins also are detergent-insoluble under these conditions, presumably due to their association with lipid rafts (Brown and London Citation1998). The phospholipid structure of GPI anchors is critical for the incorporation of GPI-anchored proteins into lipid rafts (Fujita and Jigami Citation2008) which takes place at the Golgi in mammalian cells and endoplasmic reticulum in yeast (Orlean and Menon Citation2007, Kinoshita et al. Citation2008). It is generally assumed that the long saturated fatty acyl chains typically present in the GPI phospholipid component interact with the ceramide moiety of the sphingolipids and with the cholesterol and thereby are causally involved in the lipid raft association of GPI-anchored proteins.
Consequently, it is critical for the incorporation into lipid rafts of GPI-anchored proteins that the structures of the phospholipid and glycan moieties of GPI anchors undergo extensive remodelling during biosynthesis and after attachment to the polypeptide moiety (Maeda et al. Citation2007, Fujita and Kinoshita Citation2010). Remodelling is also known to affect the transport and quality control of GPI-anchored proteins along the secretory pathway (Fujita et al. 2006b). Typically, the two fatty acyl residues are introduced into the phospholipid moiety by fatty acid remodelling at the Golgi apparatus, which is mediated by sequential lipolytic removal and reacylation of the fatty acids at the sn2 and sn1 positions by GPI-phospholipases A2/A1 (encoded by mammalian PGAP3/yeast PER1) (Fujita et al. 2006a) and lyso-GPI acyltransferases I/II (encoded by mammalian PGAP2/yeast GUP1, CWH43) (Bosson et al. Citation2006, Umemura et al. Citation2007), respectively.
Upon insertion of the hairpin-like transmembrane protein, caveolin-1, lipid rafts are thought to form vesicular invaginations, so-called caveolae (Hansen and Nichols Citation2010). Typical signalling proteins are found concentrated in lipid rafts and caveolae, which has led to the hypothesis that GPI anchors and GPI-anchored proteins located at lipid rafts and caveolae may be important in signal transduction (Müller and Frick Citation1999, Simons and Toomre Citation2000). GPI-anchored proteins can be released from plasma membrane lipid rafts and open caveolae, i.e., from the cell surface, by cleavage of the GPI anchor with serum GPI-specific phospholipase D or bacterial PI-specific phospholipase C (Hoener et al. Citation1990, Müller et al. Citation1994a, Küng et al. Citation1997).
Synthesis of plasma membrane GPI-anchored proteins
GPI anchoring of cell surface proteins is the most complex and energy-consuming lipid posttranslational modification described to date. The GPI anchor is synthesized along a membrane-bound multistep pathway in the endoplasmic reticulum requiring more than 20 gene products (Orlean and Menon Citation2007, Fujita and Kinoshita Citation2010). The pathway is initiated at the cytoplasmic leaflet of the endoplasmic reticulum and completed in its lumen, necessitating flipping of a glycolipidic intermediate across the membrane. The completed GPI anchor is attached to the protein moiety that has been translocated across the endoplasmic reticulum membrane and that is equipped with a GPI anchor signal sequence at the carboxy-terminus (Caras and Weddell Citation1989, Gerber et al. Citation1992). GPI-anchored proteins transit the classical secretory pathway via the endoplasmic reticulum and Golgi apparatus, where their incorporation into lipid rafts occurs, to the cell surface, where they remain associated with lipid rafts of the plasma membrane or, in the case of yeast, may be translocated further to the cell wall (see below). The genes encoding enzymes and transporters involved in all but one of the predicted steps in the assembly of the GPI precursor glycolipid and its transfer to the protein moiety in mammals and yeast have meanwhile been identified (Orlean and Menon Citation2007, Pittet and Conzelmann Citation2007, Kinoshita et al. Citation2008). The majority of these genes encodes polytopic membrane proteins, some of which are organized into complexes. The steps in GPI assembly, and the enzymes that carry them out, are highly conserved from yeast to man. Defects in GPI biosynthesis have been shown to cause lethality in yeast and to critically interfere with embryonic development in mammals (Leidich et al. Citation1994, Kawagoe et al. Citation1996).
For the biosynthesis of endogenous or ectopic GPI-anchored proteins, they have to be equipped with two topological signals. The amino-terminal signal sequence I guides the polypeptide precursor during co-translational transport into the endoplasmic reticulum as is typical for the majority of membrane and secretory proteins. The carboxy-terminal signal sequence II acts as a transient membrane anchor which following insertion into the endoplasmic reticulum membrane is replaced by the prefabricated GPI anchor during a coupled cleavage and transamidation reaction (Seed Citation1987, Englund Citation1993, Tiede et al. Citation1999, Orlean and Menon Citation2007). Thereafter the completed GPI-anchored protein is transported in secretory vesicles with the protein moiety residing in the vesicular lumen via the Golgi apparatus to the plasma membrane. In the course of fusion of the secretory vesicles with the Golgi apparatus, the GPI-anchored protein becomes inserted into lipid rafts of the luminal leaflet of the Golgi membrane. In polarized cells, such as epithelial and endothelial cells, both the apical and the basal plasma membrane domain can be addressed by GPI anchors, but the apical cell surface is most often targeted (Lisanti et al. Citation1989, Citation1990, Brown and Rose Citation1992).
Consequently, for ectopic expression of polypeptides for various therapeutic or biotechnological applications in appropriate (polar or non-polar) mammalian cell lines the GPI-anchored passenger proteins have to be equipped with the endoplasmic reticulum-targeting signal sequence I as well as with the hydrophobic bipartite signal sequence II (Harrison et al. Citation1994). Upon vesicular trafficking from the endoplasmic reticulum via the Golgi apparatus to the cell surface, the GPI-anchored passenger proteins are recovered with (total or plasma membrane) lipid rafts after lysis of the cells with 1% Triton X-100 in the cold and high speed-centrifugation, then extracted from the washed lipid rafts with 60 mM octylglucoside or 2% ß-amidotaurocholate (Müller et al. Citation1994b) and then purified by conventional chromatographic techniques. For certain purposes the considerable enrichment of the ectopically expressed GPI-anchored protein compared to the total cellular proteins with the prepared lipid raft materials may enable their direct use without further purification.
Synthesis of cell wall GPI-anchored proteins
The majority of cell wall proteins in fungi, such as yeast, are first modified with a GPI anchor and then become directly coupled to the cell wall ß-1,6-glucan in the course of transglycosylation of the mannose residue proximal to the glucosamine moiety or of the following mannose residue of the initial GPI anchor (Müller et al. Citation1996, Vossen et al. Citation1997, Klis et al. Citation2006) (). The protein-linked ß-1,6-glucan is cross-linked to the underlying ß-1,3-glucan network. To prove the presence of a putative formerly GPI-anchored protein in the cell wall and to assess the type of its linkage to the cell wall, the following criteria are typically used: (i) Retention of the protein in SDS-extracted cell walls (Schreuder et al. Citation1993, Cappellaro et al. Citation1994), (ii) secretion of the protein into the medium upon deletion of the carboxy-terminal 30–40 amino acids, which constitute the signal sequence II (Wojciechowicz et al. Citation1993), (iii) expression of an ectopic secretory reporter protein at the cell wall upon extension with the carboxy-terminal signal sequence II (Van Berkel et al. Citation1994), (iv) release of the protein from isolated cell walls by cleaving the phosphodiester bridge in the GPI remnant, using either HF-pyridine or a phosphodiesterase (Kapteyn et al. Citation1996, De Groot et al. Citation2005), (v) release of the protein from isolated cell walls by either a ß-1,6-glucanase or ß-1,3-glucanase or, in some special cases, by chitinase (Kollar et al. Citation1997, Fujii et al. Citation1999).
Figure 1. GPI-anchored proteins as precursors for cell wall proteins in fungi. In fungi, such as the yeast Saccharomyces cerevisiae, a special cell wall targeting (CW) signal located in proximity to the typical plasma membrane retention signal directs certain GPI-anchored proteins from the plasma membrane to the cell wall. Here they undergo direct covalent coupling to the ß-1,6-glucans in the course of enzymatic transglycosylation of the mannose residues within their core glycans and indirect cross-linking to ß-1,3-glucans, mannoproteins and chitin of the cell wall.
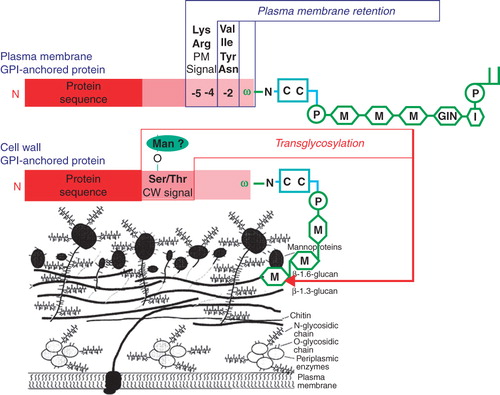
Fungal GPI-anchored proteins may be targeted to either the plasma membrane or the cell wall (Hamada et al. Citation1998, De Sampaio et al. Citation1999). An intriguing question is how the final destination of a GPI-protein is determined (Kollar et al. Citation1997, Vossen et al. Citation1997, De Sampaio et al. Citation1999). GPI-anchored proteins that are predominantly found in the plasma membrane of Saccharomyces cerevisiae generally contain two basic amino acids upstream of the GPI anchor attachment site (ω-site) (). This seems also to be the case for other fungi. Many of the predicted GPI-anchored proteins in the human pathogenic yeast strains, Candida albicans and C. glabrata are believed to be involved in adhesion and are for that reason presumably exposed to the outer surface of the cell wall (Klis et al. Citation2006). Consistently, these proteins generally lack the dibasic motif in the ω-proximal region. Instead, hydrophobic amino acids, such as valine, leucine or isoleucine, upstream of the ω-site at the ω-2, ω-4, and ω-5 positions seem to act positively to localize the protein to the cell wall (Hamada et al. Citation1998, Citation1999). A systematic mutational analysis in C. glabrata of the amino acids upstream of the ω-site has confirmed the importance of the dibasic motif for retaining GPI-anchored proteins in the plasma membrane (Frieman and Cormack Citation2003). However, the final destination of GPI-anchored proteins is not only determined by the ω-proximal region. Apparently, the presence of long serine- and threonine-rich regions, which are characteristic of many GPI-anchored proteins (), may favour targeting of a GPI-anchored protein to the cell wall and may even override the plasma membrane retention provoked by the dibasic motif in the ω-proximal region (Frieman and Cormack Citation2004).
Function
So far, no consensus role for GPI anchoring has emerged, although it is tempting to consider that the high evolutionary conservation should correlate with functional significance. There are three ways to approach this issue (Nosjean et al. Citation1997):
The GPI anchor is considered as a relic of a structure that is used to be functional under some special circumstances in the past, but that this functional significance has faded with time. Therefore, GPI anchoring would be compatible with the function of some proteins, but in fact not necessary in itself.
The power of evolution is assumed to save energy and to select biological structures and mechanisms on a functional basis. In this case, the GPI has a genuine role in cell function.
A compromise between these two options is accepted, i.e., some proteins bear the GPI anchor but could as well be anchored via a transmembrane sequence and retain their function, whereas others do require the presence of the GPI anchor to remain functional.
Functions of plasma membrane GPI-anchored proteins
Plasma membrane GPI-anchored proteins exhibit a wide diversity of functions (Low Citation1989a, 1989b, Nosjean et al. Citation1997, Ferguson Citation1999, Ikezawa Citation2002), which are apparently based on their cell surface residence, such as (T-) cell activation (e.g., Qa-2, Ly-6, DAF), complement regulation (e.g., DAF, CD59), cell-cell interactions (e.g., LFA-3) and cell migration (e.g., N-CAM, uPA), intracellular signal transduction (e.g., tyrosine phosphorylation via p59fyn, p53/p56lyn), host defence against immune attack (e.g., CD59), physical protection of parasites (e.g., Plasmodium falciparum, Trypanosoma cruzi, Toxoplasma gondii) and enzymatic activity (e.g., alkaline phosphatase, acetylcholinesterase, carbonic anhydrase, phosphodiesterase, 5′-nucleotidase). Alternatively, some functions of GPI-anchored proteins seem to be related to their import into or export from the cell, such as clathrin-independent endocytosis (e.g., PAI, folate transporter) and lipolytic release from the cell surface (e.g., alkaline phosphatase, Gce1, CD73) (Low Citation1989b, Ikezawa Citation2002, Bütikofer and Brodbeck Citation1993, Müller et al. Citation1994a, Citation1994b), translocation from plasma membrane lipid rafts onto the surface of cytoplasmic lipid droplets (e.g., Gce1, CD73) (Müller et al. Citation2008a, Citation2008b, Citation2008c, Citation2008d), or transfer from donor to acceptor cells for intercellular communication and signal transmission (e.g., Gce1, CD73) (Müller et al. Citation2009a, Citation2009b, Citation2010a, Citation2010b).
Functions of cell wall GPI-anchored proteins
The functions of fungal cell wall GPI-anchored proteins are manifold and in most cases seem to be related to cell wall porosity, flocculation, recognition of mating partners, pseudohyphal and invasive growth, iron and water retention, sterol uptake, cell wall maintenance and protection against cell wall stress, cell wall repair, adhesive properties and hydrophobicity (e.g., for polystyrene and other abiotic surfaces), cell-cell interactions, virulence, protection against oxidative stress, formation of interstitial materials and biofilms, antigenicity, iron uptake, and various enzymatic functions (De Groot et al. Citation2004, Citation2005, Klis et al. Citation2006).
Biotechnological applications
Plasma membrane GPI-anchored proteins
Production of therapeutic proteins
In principle, each soluble ectopic therapeutic protein (e.g., growth factor, hormone) or antibody can be expressed as a GPI-anchored protein at plasma membrane lipid rafts of appropriate host cells, such as yeast, insect or mammalian cells (Harrison et al. Citation1994, Shams-Eldin et al. Citation2008), from which it may be extracted by certain non-ionic detergents (Yu et al. Citation1973, Müller et al. Citation1994b, Premkumar et al. Citation2001) and further purified by conventional chromatographic procedures (see above). Alternatively, a detergent-free method for the extraction of GPI-anchored proteins which allows their simple, reliable, rapid and continuous one-step purification has been developed (Müller et al. Citation2007, and unpublished data). For this, yeast or mammalian host cells have to be immobilized on culture dishes (). Then monoclonal antibodies directed against the conserved glycan core of the GPI anchor and coupled to metallic beads are added. In the course of exposure to specifically oriented electromagnetic fields and ultrasound the GPI-anchored proteins are spontaneously extracted from the lipid rafts of the host cell plasma membrane and then stick to the magnetic plate. Subsequently the magnetic plate is removed and subjected to several washing cycles to get rid of any impurities. Finally the highly enriched and purified GPI-anchored proteins are relieved from the magnetic plate and the anti-GPI antibodies by addition of excess of competing carbohydrates. In case of using this method for the heterologous production of non-GPI-anchored proteins, the passenger protein is released from the GPI anchor by cleavage with bacterial phosphatidylinositol-specific phospholipase C or serum GPI-specific phospholipase D (Müller et al. Citation1994a, Hoener et al. Citation1990, Küng et al. Citation1997). Since this electromagnetic extraction of GPI-anchored proteins does not affect the integrity of the plasma membrane of the host cells they can be re-used after replacement of the medium ().
Figure 2. Continuous expression and one-step purification of GPI-anchored proteins by ‘electromagnetic extraction’. (A) Host cells of yeast or mammalian origin in culture dishes and stably transfected with a plasmid encoding the desired GPI-anchored protein express the GPI-anchored protein at plasma membrane lipid rafts at high abundance. (B) Antibodies directed toward the GPI anchor core glycan and coupled to magnetic beads are added. (C) A magnet is positioned at immediate neighbourhood to the cell surfaces and activated in parallel to ultrasonic treatment of the culture dishes. (D) Upon attraction of the metallic beads by the magnet, the GPI-anchored proteins are spontaneously released from the host cell lipid rafts and remain bound to the magnet. (E) The GPI-anchored proteins sticking to the magnet are washed free from any cellular and medium components. (F) The intact GPI-anchored proteins are released from the magnet by addition of excess of carbohydrates competing with the interaction between the antibodies and the GPI anchor core glycan. (G) The passenger protein moieties are released from the magnet by cleavage of the GPI anchor with bacterial phosphatidylinositol-specific phospholipase C. (H) The host cells deprived of the GPI-anchored proteins are re-used after supplementation of fresh medium.
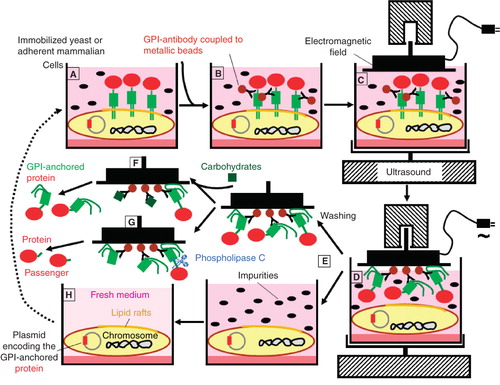
Meanwhile this detergent-free electromagnetic extraction and purification method for GPI-anchored proteins operates in continuous and automated medium-throughput mode (). In the fermentation unit A of the corresponding apparatus highly oscillating electromagnetic fields of variable strength and duration exerted by the intercalated coils cause close contact to or removal from the surface of the host cells, which express the GPI-anchored proteins, of the metallic band (, see detailed inset depicted at fermentation unit A). The oscillating movement of the metallic band together with the antibodies bound via their magnetic beads to and away from the host cell surfaces causes the interaction of the antibodies with the core glycans of the GPI-anchored proteins and in the course their extraction from the plasma membrane lipid rafts of the host cells. These remain viable and continue in the expression of new GPI-anchored proteins. The extracted GPI-anchored proteins bound to the magnetic antibodies are transported along with the metallic band from the fermentation and extraction unit A further to the harvesting and concentration unit B (). For regeneration of the antibodies directed against the glycan core in the denaturation unit C, they first have to be denatured for dissociation of the competing carbohydrates by the addition of hot SDS. The denatured and carbohydrate-less antibodies are transported along with the metallic band from the denaturation unit C further to the antibody refolding unit D. For refolding of the antibodies, appropriate folding buffer is added. After adequate incubation the refolded antibodies are transported along with the metallic band from the refolding unit D back to the fermentation and extraction unit A for the next round of extraction of the GPI-anchored proteins (). The incubation periods required for each step can be adjusted by changing the velocity of the metallic band and the dimensions of each unit. The yield obtained during each step can easily be followed with analytical devices installed between units A and B (for monitoring the efficacy of production and extraction), B and C (for monitoring the efficacy of harvesting) and C and D (for monitoring the efficacy of antibody denaturation). The media contained in each unit are filled in and removed in continuous and automatic fashion using electronically controlled pumps and valves.
Figure 3. Automated pilot device for the ‘electromagnetic extraction’. [Unit A] For fermentation and extraction, mammalian cells (green) adherent to parallel staples of plastic culture plates (red) in a tank filled with culture medium (pink) are positioned with their surfaces in immediate neighbourhood to a flexible metallic band (blue), which is continuously moved along each plate driven by a motor. Electromagnetic coils (hatched) are placed between the adjacent anti-parallel moving segments of the metallic band. Monoclonal antibodies directed against the core glycan of the GPI anchor and coupled to magnetic beads (blue circles) become bound to the metallic band denaturation. [Unit B] For harvesting the GPI-anchored proteins are displaced from the antibodies by the addition of excess of competing carbohydrates (black hexagons), which mimick the core glycan, and are then released from the GPI anchor by either enzymatic or chemical cleavage with phosphatidylinositol-specific phospholipase C (PLC) or aqueous hydrogen fluoride (HF), respectively. For subsequent concentration and removal of the GPI anchor fragments and competing carbohydrates, the buffer (yellow) containing the released and anchor-less protein moieties (red ovals) of the former GPI-anchored proteins are filtered under horizontal flow using appropriate pore size (see inset below). The retained protein moieties, i.e., the therapeutic passenger proteins or antibodies, are recovered from the filter and can be further purified if required. The antibodies together with the bound competing carbohydrates are transported along with the metallic band from the harvesting and concentration unit B further to the antibody denaturation and refolding units C and D. Their operation is explained in the text.
![Figure 3. Automated pilot device for the ‘electromagnetic extraction’. [Unit A] For fermentation and extraction, mammalian cells (green) adherent to parallel staples of plastic culture plates (red) in a tank filled with culture medium (pink) are positioned with their surfaces in immediate neighbourhood to a flexible metallic band (blue), which is continuously moved along each plate driven by a motor. Electromagnetic coils (hatched) are placed between the adjacent anti-parallel moving segments of the metallic band. Monoclonal antibodies directed against the core glycan of the GPI anchor and coupled to magnetic beads (blue circles) become bound to the metallic band denaturation. [Unit B] For harvesting the GPI-anchored proteins are displaced from the antibodies by the addition of excess of competing carbohydrates (black hexagons), which mimick the core glycan, and are then released from the GPI anchor by either enzymatic or chemical cleavage with phosphatidylinositol-specific phospholipase C (PLC) or aqueous hydrogen fluoride (HF), respectively. For subsequent concentration and removal of the GPI anchor fragments and competing carbohydrates, the buffer (yellow) containing the released and anchor-less protein moieties (red ovals) of the former GPI-anchored proteins are filtered under horizontal flow using appropriate pore size (see inset below). The retained protein moieties, i.e., the therapeutic passenger proteins or antibodies, are recovered from the filter and can be further purified if required. The antibodies together with the bound competing carbohydrates are transported along with the metallic band from the harvesting and concentration unit B further to the antibody denaturation and refolding units C and D. Their operation is explained in the text.](/cms/asset/be4ebdd4-bc50-4df8-8523-d13f2abd6a84/imbc_a_562557_f0003_b.jpg)
Interaction with membranes
A unique feature of GPI-anchored proteins is that following their extraction from cells, they are able to reintegrate into artificial membranes or plasma membranes when added to liposomes, plasma membrane vesicles or intact cells. Initial studies with sheep erythrocyte complement intermediates and the decay accelerating factor (DAF), a GPI-anchored cell surface regulator, showed that once incorporated, the exogenously added protein functioned with efficacy comparable to that of endogenous DAF protein (Medof et al. Citation1984). Subsequent studies with other GPI-anchored proteins including trypanosomal variant surface glycoprotein (Bülow et al. Citation1988), enzymes (Horta et al. Citation1991), receptors (Brodsky et al. Citation1994, Nagarajan et al. Citation1995), histocompatibility antigens (Huang et al. Citation1994), adhesion molecules (Selvaraj et al. Citation1987), as well as other immunologically relevant proteins (Zhang et al. Citation1992) have shown that, in all cases, the incorporated molecules exhibit their full native extracellular molecular interactive capabilities (Medof et al. Citation1984, Brodsky et al. Citation1994, Nagarajan et al. Citation1995) and membrane mobilities (Selvaraj et al. Citation1987, Civenni et al. Citation1998). These data established that the incorporated proteins are fully re-integrated into membrane lipid bilayers and that the incorporation is a general process attributable to the GPI moiety (Ilangumaran et al. Citation1996). Studies in which proteins with modified GPI anchor structures have been added to cells have demonstrated that stable integration and function in the membrane bilayer depends on the presence of both the sn1 and sn2 fatty acyl chains in the glycerol backbone of the attached phosphatidylinositol (Walter et al. Citation1992). A number of findings (van den Berg et al. Citation1995, Kahya et al. Citation2005, Olschewski et al. Citation2007, Sesana et al. Citation2008, Simao et al. Citation2010) suggests that added GPI-anchored proteins can associate with membranes in three ways: (i) Incompletely or otherwise incorrectly inserted, (ii) inserted as independent apparently unassociated molecules, or (iii) integrated into membrane lipid rafts, presumably as they predominantly exist in situ in cells. These data further indicate that following contact with membranes, GPI-anchored proteins undergo changes in their state of membrane attachment and that their transition into the lipid raft-associated state is a time- and temperature-dependent process.
Consequently, purified GPI-anchored proteins can be used to ‘paint’ any target cell (Medof et al. Citation1996). Such protein engineering, or ‘painting’ of cell surfaces, offers several advantages over conventional gene transfer. These advantages include: (i) GPI-anchored proteins can be painted onto cells that are difficult to transfect, (ii) cells can be altered immediately without previous culturing, (iii) the amount of GPI-anchored protein added to the surface can be precisely controlled, and (iv) multiple GPI-anchored proteins can be sequentially or simultaneously inserted into the same cells. Emerging applications for biotechnology include its use for the analysis of complex cell-surface interactions, the engineering of antigen-presenting cells and the development of cancer vaccines (Medof et al. Citation1996, Premkumar et al. Citation2001).
Transfer between cells
Since by nature the fatty acyl chains of the GPI anchor do not completely extend through the lipid bilayer, GPI-anchored proteins are associated more loosely with the plasma membrane than transmembrane proteins. In fact, very early, exogenously added purified human DAF was demonstrated to be freely mobile on the surface of sheep erythrocytes and was able to function normally as shown by its inhibition of convertase complexes (Medof et al. Citation1984). After elucidation of the structure of the GPI anchor, numerous GPI-anchored proteins have been demonstrated to be incorporated onto the surface of a variety of different cell types in vitro after their addition to intact cells (Huang et al. Citation1994, Kooyman et al. Citation1995, Anderson et al. Citation1996, McHugh et al. Citation1999). For instance, purified GPI-anchored MHC class I molecule HLA-A2.1 complexed to an antigenic peptide from hepatitis B virus was transferred to MHC-class-I-negative cells, which were then able to activate specific T-cells (Huang et al. Citation1994). Generally, the exogenously added GPI-anchored proteins retained the same characteristics and functions as the endogenously expressed GPI-anchored proteins. While the molecular mechanism underlying the transfer process is unknown, but seems to be protein-mediated rather than spontaneous (Suzuki and Okumura Citation2000), it critically depends on intact fatty acyl chains of the GPI anchor (Medof et al. Citation1996).
Transfer of GPI-anchored proteins between membranes and intact cells also can occur in vivo. Transgenic mice were engineered to express the human GPI-anchored proteins, DAF and CD59, solely on the surface of their red blood cells (Kooyman et al. Citation1995, Citation1998). Immunohistology studies with tissues from these mice detected both proteins on vascular endothelial cells from several organs, in addition to erythrocytes. Furthermore, erythrocytes from human patients with African trypanosomiasis contained membrane-bound VSG trypanosomal coat proteins (Medof et al. Citation1996). In another study, mice immunized with EG7 tumours expressing GPI-anchored B7-1 via cell surface painting, was shown to exhibit tumour-specific T-cell proliferation and cytolytic T lymphocytes (McHugh et al. Citation1995, Citation1999). These mice were protected when challenged with live wild-type tumour cells. Together the studies demonstrate that exogenously added GPI-anchored proteins spontaneously transfer from one cell to another and are functional in vivo and can potentially be used as therapeutic agents.
Transcellular transport
During the past three years it became apparent that the GPI modification can operate as a signal for transcellular transport. As is typical for eukaryotic cells, adipocytes express certain GPI-anchored proteins at their cell surface inserted into plasma membrane lipid rafts (Müller et al. Citation2001). Unexpectedly, it was found that in primary and cultured rat and mouse adipocytes, a subset of GPI-anchored proteins is associated with both plasma membrane lipid rafts (major portion) and cytoplasmic lipid droplets (minor portion) (Müller et al. Citation2008b, Citation2008d). Upon challenge of the cells with certain seemingly unrelated stimuli, such as the anti-diabetic sulfonylurea drug glimepiride, hydrogen peroxide or palmitic acid, the two GPI-anchored proteins, Gce1 and CD73, disappeared from the plasma membranes and simultaneously appeared at the cytoplasmic lipid droplets in time-dependent fashion (Müller et al. Citation2008a, Citation2008c). Surprisingly, the transport pathway of these GPI-proteins was recognized not to terminate at the surface of the lipid droplets. Aoki et al. (Citation2007) and in the following our group, too (Müller et al. Citation2009a, Citation2009b), have recently found that primary and cultured rat and mouse adipocytes release small microvesicles. In addition to a subset of adipocyte protein and lipid components, these microvesicles harboured the GPI-anchored proteins, Gce1 and CD73, already after basal release from the cells. However, their incorporation into the secreted microvesicles was strongly upregulated in response to glimepiride, hydrogen peroxide and palmitate. Thus two transport steps apparently act in concert to mediate the transcellular transport of a subset of GPI-anchored proteins from the cell surface via cytoplasmic lipid droplets into the secreted microvesicles (Müller et al. Citation2009a, Citation2009b). Interestingly, most mammalian cell types are known to release microvesicles with GPI-anchored proteins incorporated either by exocytosis, i.e., so-called exosomes (Stoorvogel et al. Citation2002, Fevrier and Raposo Citation2004, Keller et al. Citation2006) or by plasma membrane shedding, i.e., microparticles (Piccin et al. Citation2007, Cocucci et al. Citation2009). So far, a function of microvesicles in intercellular information transfer for the control of a variety of cellular functions has been amply documented (Müller Citation2011).
Very recent experiments performed with GPI-anchored and immunogold-labelled human insulin and insulin-free diabetic rats suggest that transcellular transport of GPI-anchored proteins is also operating in non-adipose tissue cells, such as enterocytes, as was deduced from electron microscopy of intestinal sections and measurements of plasma insulin and blood glucose levels (). In conclusion, GPI-anchored insulin was apparently transported from the intestinal lumen across the enterocytes into the circulation and upon distribution to the liver, muscle and adipose tissues and release from the GPI anchor through cleavage by serum phospholipases triggered insulin signalling resulting in blood glucose decrease (Müller Citation2010a, Citation2010b). No doubt, these findings should support the engineering of novel therapeutic proteins and antibodies with the crucial advantages of oral administration, selective targeting to and into the relevant diseased target cells and curing the defects in the underlying susceptibility gene products with favourable pharmacokinetic and pharmacodynamic profiles (Müller Citation2010b).
Figure 4. Hypothetical models for the transport of therapeutic RNA's and oral non-viral gene therapy on the basis of GPI modification. (Left part) Therapeutic si/microRNAs coupled to GPI anchors associate with lipid rafts of the apical plasma membrane of enterocytes following their oral administration and subsequently are transported via lipid droplets further to the cytoplasm or nucleus, where gene expression is modified accordingly. (Right part) ‘Oral’ transfection of enterocytes with the naked DNA encoding the therapeutic GPI-anchored protein under the control of appropriate 5′- and 3′-regulatory elements and surrounded by an acid-stable biofilm (grey) could be achieved with the so-called ‘gene pill’. The subsequent journey of the GPI-anchored proteins from the apical plasma membrane via caveolae, lipid droplets and microvesicles to the plasma is described in the text.
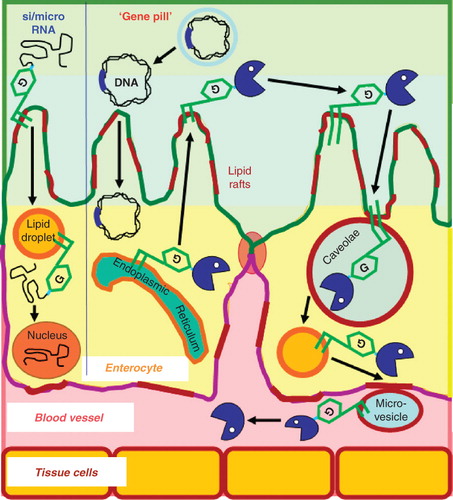
Oral gene therapy
In addition to the transport of therapeutic proteins and antibodies, the GPI modification could also mediate the oral delivery, tissue-specific targeting and cellular penetration of therapeutic nucleic acids (Xie et al. Citation2006, Kim and Rossi Citation2007, Martin and Caplen Citation2007, Gao and Huang Citation2009). Currently we are in the process of coupling specific siRNA and microRNA molecules directed against type II diabetes susceptibility genes to the GPI anchor by standard chemical crosslinking procedures. It will be interesting to see whether these constructs are taken up by the enterocytes and then transported via cytoplasmic lipid droplets to the cytoplasm and/or into the nucleus for specific, efficient and stable downregulation of the expression of the corresponding target gene product (). Alternative systems for the efficient cellular delivery of therapeutic siRNA have been reported previously, among them polyelectrolyte complex micelles of six-arm polyethylene conjugates of the siRNA and a cell-penetrating peptide with a crosslinked fusogenic peptide (Choi et al. Citation2010). In general, GPI modification could enlarge our currently rather limited repertoire of subcellular targeting strategies for drug design and delivery (Rajendran et al. Citation2010).
Moreover, GPI modification may open the possibility for oral non-viral gene therapy. The US BioTech company Genteric (Alameda, California, USA) has previously demonstrated the efficient and reliable uptake of ‘naked’ DNA, the so-called gene pill, by enterocytes in cell culture and in vivo (rats and mice) leading to marked expression and secretion into the intestinal lumen of the corresponding vector-encoded secretory gene products, e.g., growth hormone and insulin (Niedzinski et al. Citation2003, Sheu et al. Citation2003) (). Possibly, the underlying molecular mechanisms involve caveolae-mediated endocytosis as has been elucidated for the promotion of uptake of plasmid DNA and efficient gene expression by poly(glycoamidoamine) vehicles (McLendon et al. Citation2010). In case of the ‘oral transfection’ with vectors encoding genes for therapeutic GPI anchored-proteins or antibodies, their expression in enterocytes should result in the incorporation into the outer leaflet of the apical plasma membrane lipid rafts (Brown and Rose Citation1992, Fivaz et al. Citation2002). From there, i.e., from the cell surface, the described transcellular transport route across the enterocytes via caveolae and lipid droplets into the circulation presumably bound to secreted microvesicles might be initiated ().
Figure 5. The ‘gene pill’ concept. The distinct steps (1–3) of the DNA transfer via the ‘gene pill’ which consists of the relevant passenger protein cDNA sequence flanked by appropriate regulatory elements and enclosed by an acid-stable biofilm (grey) into polar enterocytes are depicted with (1) degradation of the biofilm and (2) absorption of the DNA by the enterocytes. Following expression the passenger protein is secreted from the apical plasma membrane into the intestinal lumen and then delivered across the intestinal barrier via transcellular or paracellular transport into the underlying blood vessels (3). Alternatively, the passenger protein is secreted from the basolateral plasma membrane directly into the blood vessels (4).
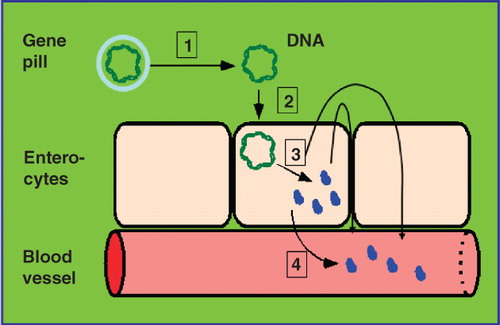
In conclusion, the ectopic expression of the GPI-anchored protein at the endoplasmic reticulum and subsequent transport to lipid rafts of the apical plasma membrane should initiate the same sequence of events as delineated previously for orally administered GPI-anchored insulin (Müller Citation2010a, 2010b). These may encompass internalization by caveolae, subsequent translocation to the surface of lipid droplets and finally transfer from the enterocytes into microvesicles. Upon release from the microvesicles by serum phospholipase action the passenger proteins or antibodies could exert their therapeutic action in the plasma or be further transported to relevant target tissues.
So far this complex journey of ectopically expressed GPI-anchored proteins, which starts at enterocytes after transient transfection with the ‘gene pill’, is pure speculation and remains to be demonstrated both in vitro and in vivo. In case of operation of this path, a number of critical points have to be clarified before clinical development may be initiated, such as the stability and variability of the gene expression, the choice of appropriate non-viral vectors, the gastric degradation of the DNA, the formulation, the gene dosage precision and the optimal time-action profile. However, the putative benefits of non-viral gene therapy, in general (Li and Huang Citation2007), and of its oral version, in particular, with its tightly regulated, specific, reversible and short-acting control of gene expression on basis of the rapid and efficient turnover of human enterocytes in vivo (and therefore presumably restriction to acute therapy) will justify the required future experimentation and expenditure.
In addition to the putative use for oral therapy, the use of GPI-modified siRNAs, microRNAs or neutralizing antibodies may be helpful for the rapid and reliable validation of putative drug targets both in vitro and in vivo. So far target validation is frequently based on the use of small molecule inhibitors or antagonist which, however, typically suffer from their unknown to limited (at best) specificity toward the enzyme or receptor target envisaged. In contrast, the introduction of siRNAs, microRNAs or neutralizing antibodies into the cytoplasm or nucleus of the relevant target cells in the course of their GPI anchor-mediated transport across the plasma membrane will lead to highly specific inhibition of expression or activity, respectively, of the putative target protein. This technology could supplement our currently available tool box for the state-of-the-art validation of novel pharmacological drug targets relying on the in vitro incubation of primary or cultured target cells or on the oral administration to relevant animal models of the disease.
Cell wall GPI-anchored proteins
Multiplex protein chips
The specific cell wall targeting information contained in certain yeast GPI anchors was used to construct protein chips for the analytics of a subset of small and large molecular weight biomarkers, such as serum metabolites (e.g., fatty acids) and proteins (e.g., adipokines). These yeast-based protein chips are based on either immobilized recombinant whole yeast cells or cell wall fragments derived thereof (Müller et al. Citation2007). These ‘natural’ and ‘custom-made’ protein chips turned out to have a number of significant advantages compared to conventional commercially available ones, such as the simultaneous measurement of multiple analytes contained in the samples per well and their simple, rapid and cheap ‘self-made’ production ().
Figure 6. GPI-protein chip vs. conventional protein chip. For the construction of GPI-protein chips appropriate recombinant receptors, binding-proteins or antibodies are simultaneously expressed as GPI-anchored cell wall proteins in yeast cells upon transfection with several multicopy plasmids. The GPI-protein chips are prepared by immobilization of either whole yeast cells or cell wall fragments derived thereof using chemical crosslinking. The techniques for reading out the bound ligands (i.e., sample analytes) by the CCD camera are similar to conventional protein chips. Conventional protein chips and GPI-protein chips share a number of advantages, with additional strengths of the latter vs. the former.
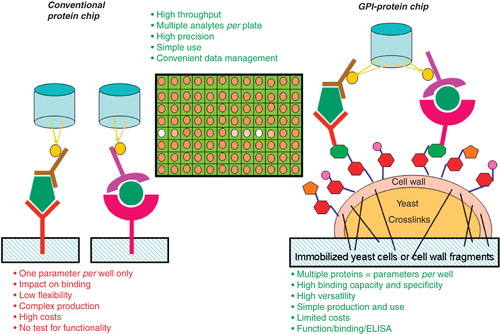
Meanwhile this GPI-anchored protein chip has been successfully configurated into a continuous and automated medium-throughput mode and is currently being evaluated for proteomic, lipidomic and metabolomic analyses on the basis of its versatile and ‘custom-made’ equipment with specific polypeptide-, lipid- and metabolite-binding proteins, receptors or antibodies (). For this the regenerated chip is loaded with excess of fluorescently (as shown here as yellow circles) or radioactively labelled analytes. After removal of free labelled analytes by repeated washing cycles and online measurement of the radioactivity or fluorescence (as shown here) signal, the samples containing the unlabelled analytes are filled into the wells. Subsequently, the displaced labelled analytes are removed by several washing cycles. The radioactivity or fluorescence (as shown here as yellow arrows) signal is measured. Thereafter the GPI-anchored proteins are denaturated by the addition of hot SDS in order to provoke the release of the analytes, which are then removed by several washing cycles. Finally, the GPI-anchored receptor, binding-protein or antibody is refolded by the addition of refolding buffer for regeneration and re-use for the next run (). The amounts of analytes contained in the sample are calculated from the differences between the two fluorescence or radioactivity signals measured for each analyt. In case of an enzyme as the analyt its activity rather than amount associated with the appropriate GPI-anchored binding-protein can be measured.
Figure 7. Automated pilot device of the GPI-protein chip. The principle is depicted for a single GPI-anchored receptor, binding-protein or antibody per well, only. The pilot device enables the simultaneous online measurement of up to five analytes (through expression of five distinct GPI-anchored proteins) per well for 12 different samples per cycle. The sequence of the individual steps per cycle are shown for the RIA/ELISA mode of operation and explained in detail in the text.
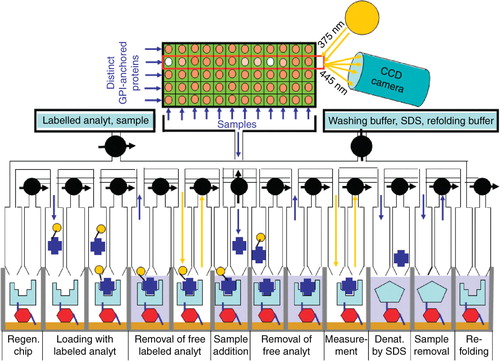
Multiplex biotransformations
The controlled and simultaneous expression of multiple cell wall GPI-anchored proteins at the surface of fungi, such as Saccharomyces Cerevisiae, offers the possibility for the straight-forward construction of novel cell-based ‘bioreactors’ for the synthesis or degradation of diverse macromolecules. As representative example the sequential multi-step hydrolysis of triacylglycerol to fatty acids and glycerol is presented (). The complete degradation of triacylglycerol embedded in lipid droplets requires three consecutive lipolytic cleavages involving adipocyte triglyceride lipase (ATGL, for the generation of diacylglycerol), hormone-sensitive lipase (HSL, for the generation of 2-monoacylglycerol) and finally 2-monoacylglycerol lipase (MAGL, for the generation of fatty acids and glycerol) (Zechner et al. Citation2005, Ahmadian et al. Citation2009). Operation of the corresponding ‘cell-based’ bioreactor depends on the simultaneous expression of all three lipases as GPI-anchored passenger proteins encoded by multi-copy plasmids under the control of constitutive and strong promoters. These are equipped with the appropriate amino-terminal signal sequence I (for cotranslational transport into the endoplasmic reticulum) and carboxy-terminal signal sequence II (for coupling to the GPI anchor), which flank the corresponding cDNA sequences coding for ATGL, HSL and MAGL (). In addition, for expression as cell wall GPI-anchored protein (here ATGL and MAGL), a cell wall signal has to be incorporated between the passenger protein cDNA and signal sequence II, which triggers the transglycosylation between the GPI anchor glycan core and the cell wall ß-1,6-glucan (see ). For expression of the plasma membrane GPI-anchored protein (here HSL), the corresponding cDNA construct lacks the cell wall signal and therefore drives the constitutive incorporation into plasma membrane lipid rafts (). The optimal topological distribution of the passenger proteins between the cell wall and the plasma membrane lipids rafts, i.e., the degree of their interaction within the periplasm and cell wall network, depends on the biochemistry of the reaction cascade (e.g., substrate availability and exchange, reaction milieu, feedback inhibitory mechanisms) envisioned.
Figure 8. Engineering of microorganisms with novel catalytic surface functions based on GPI-anchored cell wall and plasma membrane proteins. Yeast cells express enzymes for a synthesis or degradation cascade, here the sequential cleavage of triacylglycerol by adipocyte triglyceride lipase (ATGL) to diacylglycerol by hormone-sensitive lipase (HSL) to 2-monoacylglycerol by 2-monoacylglycerol lipase (MAGL) to fatty acids and glycerol. Upon covalent coupling of the cell wall GPI-anchored proteins (here ATGL and MAGL) to the ß-1,6-glucans and insertion of the GPI-anchored plasma membrane protein into lipid rafts (here HSL) the complete enzymic cascade (here the degradation of lipid droplets) becomes operative at the interface between the cell wall and the plasma membrane (i.e., within the periplasm).
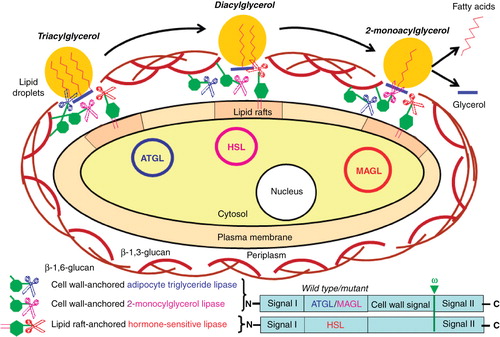
For triacylglycerol degradation, localization of both ATGL and MAGL at the cell wall and of HSL at plasma membrane lipid rafts turned out to lead to the highest turnover rates of triacylglycerol into fatty acids and glycerol. The close spatial proximity of the different enzymes and substrates involved at the interface between the cell wall and the periplasm may significantly contribute to the increased efficacy and specificity of the overall biotransformation. The underlying molecular mechanism remains to be elucidated but may be related to unhindered access of the exogenously added lipid droplets to cell wall-associated ATGL as well as of the generated diacylglycerol to lipid raft-associated HSL in combination with the missing feedback inhibition of HSL by the generated 2-monoacylglycerol due to rapid cleavage by cell wall-associated MAGL. Moreover, for equivalent yields in the desired end product the recombinant production of yeast cells is less demanding in time and costs compared to that of multiple purified enzymes. These findings exemplify the multifold advantages of this novel type of multiplex biotransformation compared to conventional ‘single-compartment’ cell-free or cell-based reaction cascades. A huge array of other biotransformations are conceivable, among them the convenient and rapid combinatorial synthesis of novel macromolecules or small molecules on basis of multiple reaction cascades with wild-type or mutant biosynthetic enzymes. Taken together, combinatorial biotransformations based on GPI-anchored plasma membrane and cell wall proteins may significantly increase the variety of synthesis and degradation pathways and, in consequence, the structural divergence of molecules relevant for applied red and white biotechnology.
Nanoparticles
The amphiphilic nature of the GPI anchor imposes novel biophysical characteristics to the coupled protein moiety with regard to adherence to hydrophobic and amphiphilic surfaces and materials. As a consequence purified GPI-anchored proteins can be reconstituted into mixed micelles, liposomes (Nosjean and Roux Citation1999, Citation2003, Morandat et al. Citation2002, Ronzon et al. Citation2004), model lipid rafts (Milhiet et al. Citation2002), or lipid droplets harbouring a core of neutral triacylglycerols (Müller et al. Citation2008b, Citation2008d) as well as be adsorbed to natural and artificial polymers (). In addition, GPI-anchored proteins converted into fungal cell wall proteins as described above (see ) can be crosslinked to each other by chemical means or covalently linked to biomaterials, which constitute the shell of the nanoparticles. These diverse assemblies, aggregates and ‘bubbles’ with grossly differing properties are currently being evaluated for a number of different purposes, among them the transport across intestinal enterocytes.
Figure 9. Assembly of GPI-anchored proteins into nanoparticles. GPI-anchored plasma membrane proteins can be inserted into detergent/phospholipid micelles, liposomes with preferential reconstitution at the surface rather than in the lumen and lipid droplets with reconstitution at the surface phospholipid monolayer. Cell wall GPI-anchored proteins with bound ß-1,6-glucans can be covalently crosslinked to each other. These aggregates are encapsulated by shells formed from biomaterials. Alternatively, cell wall GPI-anchored proteins can be directly coupled to the shell of so-called bubbles.
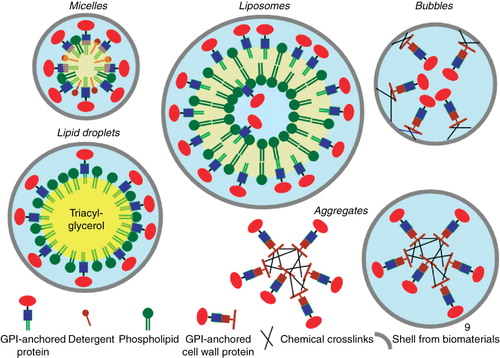
So far the long path from the mouth to the drug target within the body across the intestinal and cellular barriers has remained the major hurdle for the use of protein therapeutics in humans. The intestine is lined by a monolayer of epithelial cells, so-called enterocytes, which are interconnected to each other by specific protein complexes, so-called tight junctions (see ). Macromolecules, such as proteins and nucleic acids, typically cannot cross the tight junctions for the so-called paracellular passage from the intestinal lumen directly into the circulation. However, paracellular transport of protein drugs by encapsulation into nanoparticles assembled from various biomaterials, such as chitosan, alginate, mannans and γ-polyglutamic acid at specific ratios, has been demonstrated (Mathiowitz et al. Citation1997, Lin et al. Citation2007, Mathiowitz Citation2008). These nanoparticles apparently adhere to the negatively charged mucus and glycocalyx layers of the small intestine, which is achieved by their positive net surface charge. Following infiltration of these layers the nanoparticles trigger opening of the tight junctions, presumably by causing the redistribution of some of their constituent proteins, e.g., F-actin and ZO-1, for their subsequent passage between neighbouring enterocytes. However, immediately thereafter the tight junctions reseal again in order to maintain the intestinal permeability barrier (Mathiowitz et al. Citation1997).
Albeit these initial findings are promising, many critical requirements for (chronic) use in humans, predominantly concerning safety, non-immunogenicity and non-accumulation in the body due to their proper degradation and excretion, have to be met by these nanoparticles. So far, materials fulfilling all these criteria are not available and consequently there is an urgent need for the discovery and development of novel biomaterials. Initial results with mixed micelles consisting of phosphatidic acid, cholesterol, negatively charge glycosphingolipids and GPI-anchored therapeutic proteins, such as human insulin, and encapsulated together with some of the above biomaterials could represent a major advantage in oral protein delivery. When assayed in vitro for paracellular transport (Müller Citation2010a, Citation2010b) these nanoparticles were shown to trigger the opening and closure of the tight junctions in reversible and efficient fashion, presumably due to the amphiphilic overall nature exerted by the GPI anchor. Moreover, when enwrapped by an acid-stable film these nanoparticles have demonstrated their potential to increase plasma insulin and lower blood glucose in insulin-free diabetic rats. Importantly, during these preliminary studies accumulation of these nanoparticles or of their degradation products and other safety issues were not observed, possibly due to efficient elimination and excretion of all the biomaterials used, including the GPI anchor. The pharmacokinetic/dynamic challenges, such as the adjustment of the time-action profile and the control of the interindividual variability of the anchor removal, will be similar for the paracellular and transcellular (see above) routes of oral protein delivery on the basis of GPI-anchored therapeutic proteins, but do not seem to represent insurmountable hurdles for the future (Müller Citation2010b).
Furthermore, biodegradable nanoparticles harbouring GPI-anchored proteins have recently been used as an adjuvant by targeting antigens to antigen-presenting cells, such as dendritic cells and macrophages (Bumgarner et al. Citation2009). Here the two immunostimulatory proteins, B7-1 and ICAM-1, were attached via their engineered GPI anchors simultaneously to hydrophobic pockets at the surface of nanoparticles composed of fatty acid-binding albumin. The demonstrated functional activity and stability during storage of these complexes of GPI-anchored B7-1 and ICAM-1 suggest that albumin nanoparticles with functional GPI-anchored receptors or binding-proteins exposed at their surface may have the potential for operation as delivery devices for antigens in vaccines or for the controlled tissue targeting and distribution of protein and nucleic acid drugs upon their inclusion into the hydrophilic lumen of these nanoparticles (Bumgarner et al. Citation2009).
Conclusions
There may have been good reasons for nature to introduce GPI-anchored proteins during the evolution of eukaryotic cells, albeit we currently do not understand their functional advantages compared to more frequently realized types of membrane anchorage very well. There may be even better reasons to use GPI-anchored proteins in pharmaceutical and industrial biotechnology on basis of their unique biophysical and cell biological properties. At present, protein chips for multiplex analytics and novel biomaterials for multi-step catalysis as well as therapeutic proteins for transport into relevant target cells and/or oral administration seem to be most promising for taking advantage of the GPI anchorage of passenger polypeptides to fungal cell walls, liposomes, nanoparticles or other assemblies. The time is now ripe for the production of GPI-anchored plasma membrane and cell wall proteins for the plethora of biotechnological applications.
Acknowledgements
The author would like to thank the two reviewers for very valuable comments and suggestions which were all considered for the final version of the text with considerable gain in information.
Declaration of interest: The author reports no conflicts of interest. The author alone is responsible for the content and writing of the paper. The author is currently employee of Sanofi-Aventis Deutschland GmbH.
References
- Ahmadian M, Duncan RE, Sul HS. 2009. The skinny on fat: Lipolysis and fatty acid utilization in adipocytes. Trends Endocrinol Metab 20:424–428.
- Anderson SM, Yu G, Giattina M, Miller JL. 1996. Intercellular transfer of a glycosylphosphatidylinositol (GPI)-linked protein: Release and uptake of CD4-GPI from recombinant adeno-associated virus-transduced HeLa cells. Proc Natl Acad Sci USA 93:5894–5898.
- Aoki N, Jin-no S, Nakagawa Y, Asai N, Arakawa E, Tamura N. 2007. Identification and characterization of microvesicles secreted by 3T3-L1 adipocytes: Redox- and hormone-dependent induction of milk fat globule-epidermal growth factor 8-associated microvesicles. Endocrinology 148:3850–3862.
- Bosson R, Jaquenoud M, Conzelmann A. 2006. GUP1 of Saccharomyces cerevisiae encodes an O-acyltransferase involved in remodeling of the GPI anchor. Mol Biol Cell 17:2636–2645.
- Brewis IA, Ferguson MAJ, Mehlert A, Turner AJ, Hooper NM. 1995. Structures of the glycosyl-phosphatidylinositol anchors of porcine and human eythrocyte renal membrane dipeptidase. Comprehensive structural studies on the porcine anchor and interspecies comparison of the glycan core structures. J Biol Chem 270:22946–22956.
- Brodsky RA, Jane SM, Medof ME, Vanin EC, Shimada T, Peters TR, Nienhus AW. 1994. Purified CD4-DAF can incorporate into CD4- cells and function as a receptor for targeted HIV-mediated gene transfer. Human Gene Therapy 5:1231–1239.
- Brown DA. 1992. Interactions between GPI-anchored proteins and membrane lipids. Trends Cell Biol 2:338–343.
- Brown DA, London L. 1998. Functions of lipid rafts in biological membranes. Annu Rev Cell Dev Biol 14:111–136.
- Brown DA, Rose JK. 1992. Sorting of GPI-anchored proteins to glycolipid-enriched membrane subdomains during transport to the apical cell surface. Cell 68:533–544.
- Bumgarner GW, Shashidharamurthy R, Nagarajan S, D'souza MJ, Selvaraj P. 2009. Surface engineering of microparticles by novel protein transfer for targeted antigen/drug delivery. J Control Release 137:90–97.
- Bülow R, Overath P, Davoust J. 1988. Rapid lateral diffusion of the variant surface glycoprotein in the coat of Trypanosoma brucei. Biochemistry 27:2384–2388.
- Bütikofer P, Brodbeck U. 1993. Partial purification and characterization of a (glycosyl) inositol phospholipid-specific phospholipase C from peanut. J Biol Chem 268:17794–17799.
- Cappellaro C, Baldermann C, Rachel R, Tanner W. 1994. Mating type-specific cell-cell recognition of Saccharomyces cerevisiae: Cell wall attachment and active sites of a- and α-agglutinin. EMBO J 13:4737–4744.
- Caras IW, Weddell GN. 1989. Signal peptide for protein secretion directing glycophospholipid membrane anchor attachment. Science 243:1196–1198.
- Choi SW, Lee SH, Mok H, Park TG. 2010. Multifunctional siRNA delivery system: Polyelectrolyte complex micelles of six-arm PEG conjugate of siRNA and cell penetrating peptide with crosslinked fusogenic peptide. Biotechnol Prog 26:57–63.
- Civenni G, Test ST, Brodbeck U, Bütikofer P. 1998. In vitro incorporation of GPI-anchored proteins into human erythrocytes and their fate in the membrane. Blood 9:11784–11792.
- Cocucci E, Racchetti G, Meldolesi J. 2009. Shedding microvesicles: Artefacts no more. Trends Cell Biol 19:43–51.
- Deeg MA, Humphrey DR, Yang SH, Ferguson TR, Reinhold VN, Rosenberry TL. 1992. Glycan components in the glycoinositol phospholipid anchor of human erythrocyte acetylcholinesterase. Novel fragments produced by trifluoroacetic acid. J Biol Chem 267:18573–18580.
- De Groot PWJ, De Boer AD, Cunningham J. 2004. Proteomic analysis of Candida albicans cell walls reveals covalently bound carbohydrate-active enzymes and adhesins. Eukaryot Cell 3:955–965.
- De Groot PWJ, Ram AF, Klis FM. 2005. Features and functions of covalently linked proteins in fungal cell walls. Fungal Genetics Biol 42:657–675.
- De Sampaio G, Bourdineaud J-P, Laquin J-M. 1999. A constitutive role for GPI anchors in Saccharomyces cerevisiae cell wall targeting. Mol Microbiol 150:3105–3114.
- Englund PT. 1993. The structure and biosynthesis of glycosyl phosphatidylinositol protein anchors. Annu Rev Biochem 62:121–139.
- Ferguson MA. 1999. The structure, biosynthesis and functions of glycosylphosphatidylinositol anchors, and the contributions of trypanosoma research. J Cell Sci 112:2799–2809.
- Ferguson MA, Low MG, Cross GA. 1985. Glycosyl-sn-1,2-dimyristylphosphatidylinositol is covalently linked to Trypanosoma brucei variant surface glycoprotein. J Biol Chem 260:14547–14555.
- Fevrier B, Raposo G. 2004. Exosomes: Endosomal-derived vesicles shipping extracellular messages. Curr Opin Cell Biol 16:415–421.
- Fivaz M, Vilbois F, Thurnheer S, Pasquali C, Abrami L, Bickel PE, Parton RG, van der Goot FG. 2002. Differential sorting and fate of endocytosed GPI-anchored proteins. EMBO J 21:3989–4000.
- Frieman MB, Cormack BP. 2003. The omega-site sequence of glycosylphosphatidylinositol-anchored proteins in Saccharomyces cerevisiae can determine distribution between the membrane and the cell wall. Mol Microbiol 50:883–896.
- Frieman MB, Cormack BP. 2004. Multiple sequence signals determine the distribution of glycosylphosphatidylinositol proteins between the plasma membrane and cell wall in Saccharomyces cerevisiae. Microbiology 150:3105–3114.
- Fujii T, Shimoi H, Iimura Y. 1999. Structure of the glucan-binding sugar chain of Tip1p, a cell wall protein of Saccharomyces cerevisiae. Biochim Biophys Acta 1427:133–144.
- Fujita M, Jigami Y. 2008. Lipid remodeling of GPI-anchored proteins and its function. Biochim Biophys Acta 1780:410–420.
- Fujita M, Kinoshita T. 2010. Structural remodeling of GPI anchors during biosynthesis and after attachment to proteins. FEBS Lett 584:1670–1677.
- Fujita M, Umemura M, Yoko OT, Jigami Y. 2006a. PER1 is required for GPI-phospholipase A2 activity and involved in lipid remodeling of GPI-anchored proteins. Mol Biol Cell 17:5253–5264.
- Fujita M, Yoko OT, Jigami Y. 2006b. Inositol deacylation by Bst1p is required for the quality control of glycosylphosphatidylinositol-anchored proteins. Mol Biol Cell 17:834–850.
- Gao K, Huang L. 2009. Nonviral methods for siRNA delivery. Mol Pharmaceut 6:651–658.
- Gerber LD, Kodukula K, Udenfriend, S. 1992. Phosphatidylinositol glycan (PI-G) anchored membrane proteins: Amino acid requirements adjacent to the site of cleavage, and PI-G attachment in the COOH-terminal signal peptide. J Biol Chem 267:12168–12173.
- Hamada K, Terashima H, Arisawa M, Kitada K. 1998. Amino acid sequence requirements for efficient incorporation of glycosylphosphatidylinositol-associated proteins into the cell wall of Saccharomyces cerevisiae. J Biol Chem 273:26946–26953.
- Hamada K, Terashima H, Arisawa M, Yabuki N, Kitada K. 1999. Amino acid residues in the omega-minus region participate in cellular localization of yeast glycosylphosphatidylinositol-attached proteins. J Bacteriol 181:3886–3889.
- Hansen CG, Nichols BJ. 2010. Exploring the caves: Cavins, caveolins and caveolae. Trends Cell Biol 20:177–186.
- Harrison PT, Hutchinson MJ, Allen JM. 1994. A convenient method for the construction and expression of GPI-anchored proteins. Nucleic Acids Res 22:3813–3814.
- Hoener MC, Stieger S, Brodbeck U. 1990. Isolation and characterization of a phosphatidylinositol-glycan-anchor-specific phospholipase D from bovine brain. Eur J Biochem 190:593–600.
- Homans SW, Ferguson MA, Dwek RA, Rademacher TW, Anand R, Williams AF. 1985. Complete structure of the glycosyl phosphatidylinositol membrane anchor of rat brain Thy-1 glycoprotein. Nature 333:269–272.
- Horta MF, Ramalho-Pinto FJ, Horta MF. 1991. Role of human deacy-accelerating factor in the evasion of Schistosoma mansoni from the complement-mediated killing in vitro. J Exp Med 174:1399–1406.
- Huang JH, Getty RR, Chisari FV, Fowler P, Greenspan NS, Tykocinski ML. 1994. Protein transfer of preformed MHC-peptide complexes sensitizes target cells to T cell cytolysis. Immunity 1:607–613.
- Ilangumaran S, Robinson PJ, Hoessli DC. 1996. Transfer of exogenous glycosylinositol (GPI)-linked molecules to plasma membranes. Trends Cell Biol 6:163–169.
- Kahya N, Brown DA, Schwille P. 2005. Raft partitioning and dynamic behavior of human placental alkaline phosphatase in giant unilamellar vesicles. Biochemistry 24:7479–7489.
- Kapteyn JC, Montijn RC, Vink E. 1996. Retention of Saccharomyces cerevisiae cell wall proteins through a phosphodiester-linked ß-1,3-/ß-1,6-glucan heteropolymer. Glycobiology 6:337–345.
- Kawagoe K, Kitamura D, Okabe M, Taniuchi I, Ikawa M, Watanabe T, Kinoshita T, Takeda J. 1996. Glycosylphosphatidylinositol-anchor-deficient mice: Implications for clonal dominance of mutant cells in paroxysmal nocturnal hemoglobinuria. Blood 87:3600–3606.
- Keller S, Sanderson MP, Stoeck A, Altevogt P. 2006. Exosomes: From biogenesis and secretion to biological function. Immunol Lett 107:102–108.
- Kim DH, Rossi JJ. 2007. Strategies for silencing human disease using RNA interference. Nat Rev Genet 8:173–184.
- Kinoshita T, Fujita M, Maeda Y. 2008. Biosynthesis, remodelling and functions of mammalian GPI-anchored proteins: Recent progress. J Biochem 144:287–294.
- Klis FM, Boorsma A, De Groot PW. 2006. Cell wall construction in Saccharomyces cerevisiae. Yeast 23:185–202.
- Kollar R, Reinhold BB, Petrakova E, Yeh HJ, Ashwell G, Drgonova J, Kapteyn JC, Klis FM, Cabib E. 1997. Architecture of the yeast cell wall. ß(1-6)-glucan interconnects mannoprotein, ß(1-3)-glucan, and chitin. J Biol Chem 272:17762–17775.
- Kooyman DL, Byrne GW, McClellan S, Nielsen S, Tone M, Waldmann H, Coffman TM, McCurry KR, Platt JL, Logan JS. 1995. In vivo transfer of GPI-linked complement restriction factors from erythrocytes to the endothelium. Science 269:89–92.
- Kooyman DL, Byrne GW, Logan JS. 1998. Glycosyl phosphatidylinositol anchor. Exp Nephrol 6:148–151.
- Küng M, Bütikofer P, Brodbeck U, Stadelmann B. 1997. Expression of intracellular and GPI-anchored forms of GPI-specific phospholipase D in COS-1 cells. Biochem Biophys Acta 1357:329–338.
- Ikezawa H. 2002. Glyosylphosphatidylinositol (GPI)-anchored proteins. Biol Pharm Bull 25:409–417.
- Leidich SD, Drapp DA, Orlean P. 1994. A conditionally lethal yeast mutant blocked at the first step in glycosyl phosphatidylinositol anchor synthesis. J Biol Chem 269:10193–10196.
- Li SD, Huang L. 2007. Non-viral is superior to viral gene delivery. J Controlled Release 123:181–183.
- Lin YH, Mi FL, Chen CT, Chang WC, Peng SF, Liang HF, Sung HW. 2007. Preparation and characterization of nanoparticles shelled with chitosan for oral insulin delivery. Biomacromolecules 8:146–152.
- Lisanti MP, Caras IW, Davit MA, Rodriguez-Boulan E. 1989. Glycophospholipid membrane anchor acts as an apical targeting signal in polarized epithelial cells. J Cell Biol 109:2145–2156.
- Lisanti MP, Rodriguez-Boulan E, Saltiel AR. 1990. Emerging functional roles for the glycosylphosphatidylinositol membrane anchor. J Membr Biol 117:1–10.
- Low MG. 1989a. The glycosyl-phosphatidylinositol anchor of membrane proteins. Biochim Biophys Acta 988:427–454.
- Low MG. 1989b. Glycosyl-phosphatidylinositol: A versatile anchor for cell surface proteins. FASEB J 3:1600–1608.
- Maeda Y, Tashima Y, Houjou T, Fujita M, Yoko OT, Jigami Y, Taguchi R, Kinoshita T. 2007. Fatty acid remodeling of GPI-anchored proteins is required for their raft association. Mol Biol Cell 18:1497–1506.
- Martin SE, Caplen NJ. 2007. Development of new RNAi therapeutics. Histol Histopathol 22:211–217.
- Mathiowitz E. 2008. Drug delivery system. Toxicol Pathol 36:6–22.
- Mathiowitz E, Jacob JS, Jong YS, Carino GP, Chickering DE, Chaturvedi P, Santos CA, Vijayaraghavan K, Montgomery S, Bassett M, Morrell C. 1997. Biologically erodable microspheres as potential oral drug delivery systems. Nature 386:410–414.
- McConville MJ, Ferguson MA. 1993. The structure, biosynthesis and function of glycosylated phosphatidylinositols in the parasite protozoa and higher eukaryotes. Biochem J 294:305–324.
- McHugh RS, Ahmed SN, Wang YC, Sell KW, Selvavaj P. 1995. Construction, purification, and functional incorporation on tumor cells of glycolipid-anchored human B7-1 (CD80). Proc Natl Acad Sci USA 92:8059–8063.
- McHugh RS, Nagarajan S, Wang YC, Sell KW, Selvaraj P. 1999. Protein transfer of glycosyl-phosphatidylinositol-B7-1 into tumor cell membranes: A novel approach to tumor immunotherapy. Cancer Res 59:2433–2437.
- McLendon PM, Fichter KM, Reineke TM. 2010. Poly(glycoamidoamine) vehicles promote pDNA uptake through multiple routes and efficient gene expression via caveolae-mediated endocytosis. Mol Pharmaceut 7:738–750.
- Medof ME, Kinoshita T, Nussenzweig V. 1984. Inhibition of complement activation on the surface of cells after incorporation of decay accelerating factor (DAF) into their membranes. J Exp Med 160:1558–1578.
- Medof ME, Nagarajan S, Tykocinski ML. 1996. Cell-surface engineering with GPI-anchored proteins. FASEB J 10:574–586.
- Milhiet P-E, Giocondi M-C, Baghdadi O, Ronzon F, Roux B, Le Grimellec C. 2002. Spontaneous insertion and partitioning of alkaline phosphatase into model lipid rafts. EMBO Rep 3:485–490.
- Morandat S, Bortolato M, Roux B. 2002. Cholesterol-dependent insertion of glycosylphosphatidylinositol-anchored enzyme. Biochim Biophys Acta 1564:473–478.
- Munro S. 2003. Lipid rafts: Elusive or illusive? Cell 115:377–388.
- Müller G. 2010a. Oral protein therapy for the future? Transport of glycolipid-modified therapeutic proteins. Pharmacology 86:92–116.
- Müller G. 2010b. Oral delivery of protein drugs – driver of personalized medicine? Curr Issues Mol Biol 13:13–24.
- Müller G. 2011. Control of lipid storage and cell size between adipocytes by vesicle-associated glycosylphosphatidylinositol-anchored proteins. Arch Physiol Biochem 117:23–43.
- Müller G, Dearey E-A, Korndörfer A, Bandlow W. 1994a. Stimulation of a glycosyl-phosphatidylinositol-specific phospholipase by insulin and the sulfonylurea, glimepiride, in rat adipocytes depends on increased glucose transport. J Cell Biol 126:1267–1276.
- Müller G, Frick W. 1999. Signalling via caveolin: Involvement in the cross-talk between phosphoinositolglycans and insulin. Cell Mol Life Sci 56:945–970.
- Müller G, Groß E, Wied S, Bandlow W. 1996. Glucose-induced sequential processing of a glycosyl-phosphatidylinositol-anchored ectoprotein in Saccharomyces cerevisiae. Mol Cell Biol 16:442–456.
- Müller G, Jung C, Straub J, Wied S, Kramer W. 2009a. Induced release of membrane vesicles from rat adipocytes containing glycosylphosphatidylinositol-anchored microdomain and lipid droplet signalling proteins. Cell Signal 21:324–338.
- Müller G, Jung C, Wied S. 2008a. Translocation of glycosylphosphatidylinositol-anchored proteins from plasma membrane microdomains to lipid droplets in rat adipocytes is induced by palmitate, H2O2 and the sulfonylurea drug, glimepiride. Mol Pharmacol 73:1513–1529.
- Müller G, Jung C, Wied S, Biemer-Daub G. 2009b. Induced translocation of glycosylphosphatidylinositol-anchored proteins from lipid droplets to adiposomes in rat adipocytes. Br J Pharmacol 158:749–770.
- Müller G, Jung C, Wied S, Welte S, Jordan H, Frick W. 2001. Redistribution of glycolipid raft domain components induces insulin-mimetic signaling in rat adipocytes. Mol Cell Biol 21:4553–4567.
- Müller G, Korndörfer A, Saar K, Karbe-Thönges B, Fasold H, Müllner S. 1994b. 4′-Amino-benzamido-taurocholic acid selectively solubilizes glycosyl-phosphatidylinositol-anchored membrane proteins and improves lipolytic cleavage of their membrane anchors by specific phospholipases. Arch Biochem Biophys 309:329–340.
- Müller G, Over S, Wied S, Frick W. 2008b. Association of (c)AMP-degrading glycosylphosphatidylinositol-anchored proteins with lipid droplets is induced by palmitate, H2O2 and the sulfonylurea drug, glimepiride, in rat adipocytes. Biochemistry 47:1274–1287.
- Müller G, Tripier D, Müllner S. 2007. Phosphoinositolglycan-peptides and applications. European patent EP0532915.
- Müller G, Wied S, Dearey E-A, Biemer-Daub G. 2010a. Glycosylphosphatidylinositol-anchored proteins coordinate lipolysis inhibition between large and small adipocytes. Metabolism, doi:10.1016/j.metabol.2010.10.007.
- Müller G, Wied S, Jung C, Biemer-Daub G, Frick W. 2010b. Transfer of glycosylphosphatidylinositol-anchored 5′-nucleotidase CD73 from adiposomes into rat adipocytes stimulates lipid synthesis. Br J Pharmacol 160:878–891.
- Müller G, Wied S, Jung C, Over S. 2008c. Translocation of glycosylphosphatidylinositol-anchored proteins to lipid droplets and inhibition of lipolysis in rat adipocytes is mediated by reactive oxygen species. Br J Pharmacol 154:901–913.
- Müller G, Wied S, Over S, Frick W. 2008d. Inhibition of lipolysis by palmitate, H2O2 and the sulfonylurea drug, glimepiride, in rat adipocytes depends on cAMP degradation by lipid droplets. Biochemistry 47:1259–1273.
- Nagarajan S, Anderson M, Ahmed SN, Sell KW, Selvaraj P. 1995. Purification and optimization of functional reconstitution on the surface of leukemic cell lines of GPI-anchored Fc receptor III. J Immunol Meth 184:241–251.
- Niedzinski EJ, Chen Y-J, Olson DC, Parker EA, Park H, Udove JA, Scollay R, McMahon BM. 2003. Enhanced systemic transgene expression after nonviral salivary gland transfection using a novel endonuclease inhibitor/DNA formulation. Gene Ther 10:2133–2138.
- Nosjean O, Briolay A, Roux B. 1997. Mammalian GPI proteins: Sorting, membrane residence and functions. Biochim Biophys Acta 1331:153–186.
- Nosjean O, Roux B. 1999. Ectoplasmic insertion of a glycosylphosphatidylinositol-anchored protein in glycosphingolipid- and cholesterol-containing phosphatidylcholine vesicles. Eur J Biochem 263:865–870.
- Nosjean O, Roux B. 2003. Anchoring of glycosylphosphatidylinositol-proteins to liposomes. Methods Enzymol 372:216–232.
- Olschewski D, Seidel R, Miesbauer M, Rambold AS, Oesterhelt D, Winklhofer KF, Tatzelt J, Engelhard M, Becker CF. 2007. Semisynthetic murine prion protein equipped with a GPI anchor mimic incorporates into cellular membranes. Chem Biol 14:994–1006.
- Orlean P, Menon AK. 2007. Thematic review series: lipid posttranslational modifications. GPI anchoring of protein in yeast and mammalian cells, or: How we learned to stop worrying and love glycophospholipids. J Lipid Res 48:993–1011.
- Piccin A, Murphy WG, Smith OP. 2007. Circulating microparticles: Pathophysiology and clinical implications. Blood Rev 21:157–171.
- Pittet M, Conzelmann A. 2007. Biosynthesis and function of GPI proteins in the yeast Saccharomyces cerevisiae. Biochim Biophys Acta 1771:405–420.
- Premkumar DR, Fukuoka Y, Sevlever D, Brunschwig E, Rosenberry TL, Tykocinski ML. 2001. Properties of exogenously added GPI-anchored proteins following their incorporation into cells. J Cell Biochem 82:234–245.
- Rajendran L, Knölker H-J, Simons K. 2010. Subcellular targeting strategies for drug design and delivery. Nature Rev Drug Discovery 9:29–42.
- Rajendran L, Simons K. 2005. Lipid rafts and membrane dynamics. J Cell Sci 118:1099–1102.
- Ronzon F, Morandat S, Roux B, Bortolato M. 2004. Insertion of a glycosylphosphatidylinositol-anchored enzyme into liposomes. J Membrane Biol 197:169–177.
- Schreuder MP, Brekelmans S, Van den Ende H, Klis FM. 1993. Targeting of a heterologous protein to the cell wall of Saccharomcyes cerevisiae. Yeast 9:399–409.
- Seed B. 1987. An LFA-3 cDNA encodes a phospholipid-linked membrane protein homologous to its receptor CD2. Nature 313:812–815.
- Selvaraj P, Dustin ML, Silber R, Low MG, Springer TA. 1987. Deficiency of lymphocyte function-associated antigen 3 (LFA-3) in paroxysmal nocturnal hemoglobinuria. J Exp Med 166:1011–1025.
- Sesana S, Re F, Bulbarelli A, Salerno D, Cazzaniga E, Masserini M. 2008. Membrane features and activity of GPI-anchored enzymes: alkaline phosphatase reconstituted in model membranes. Biochemistry 47:5433–5440.
- Shams-Eldin H, Azzouz N, Niehus S, Smith TK, Schwarz RT. 2008. An efficient method to express GPI-anchor proteins in insect cells. Biochem Biophys Res Commun 365:657–663.
- Sheu E, Rothman S, German M, Wang X, Finer M, McMahon BM. 2003. The gene pill and its therapeutic applications. Curr Opin Mol Ther 5:420–427.
- Simao AM, Yadav MC, Narisawa S, Bolean M, Pizauro JM, Hoylaerts MF, Ciancaglini P, Millan JL. 2010. Proteoliposomes harboring alkaline phosphatase and nucleotide pyrophosphatase as matrix vesicle biomimetics. J Biol Chem 285:7598–7609.
- Simons K, Toomre D. 2000. Lipid rafts and signal transduction. Nat Rev Mol Cell Biol 1:31–39.
- Singer SJ, Nicolson GL. 1972. The fluid mosaic model of the structure of cell membranes. Science 175:720–728.
- Stoorvogel W, Kleijmeer MJ, Geuze H, Raposo G. 2002. The biogenesis and functions of exosomes. Traffic 3:321–330.
- Suzuki K, Okumura Y. 2000. GPI-linked proteins do not transfer spontaneously from erythrocytes to liposomes. New aspects of reorganization of the cell membrane. Biochemistry 39:9477–9485.
- Tiede A, Bastisch I, Schubert J, Orlean P, Schmidt RE. 1999. Biosynthesis of glycosylphosphatidylinositols in mammals and unicellular microbes. Biol Chem 380:503–523.
- Umemura M, Fujita M, Yoko OT, Fukamizu A, Jigami Y. 2007. Saccharomyces cerevisiae CWH43 is involved in remodeling of the GPI anchor. Mol Biol Cell 18:4304–4316.
- Van Berkel MAA, Caro LHP, Montijn RC, Klis FM. 1994. Glucosylation of chimeric proteins in the cell wall of Saccharomyces cerevisiae. FEBS Lett 349:135–138.
- van den Berg CW, Cinek T, Hallett MB, Horejsi V, Morgan BP. 1995. Exogenous glycosyl phosphatidylinositol-anchored CD59 associates with kinases in membrane clusters on U937 cells and becomes Ca2+-signaling competent. J Cell Biol 131:669–677.
- Varma R, Mayor S. 1998. GPI-anchored proteins are organized in submicron domains at the cell surface. Nature 394:798–801.
- Vossen JH, Müller WH, Lipke PN, Klis FM. 1997. Restrictive glycosylphosphatidylinositol anchor synthesis in cwh6/gpi3 yeast cells causes aberrant biogenesis of cell wall proteins. J Bacteriol 179:2202–2209.
- Walter EI, Ratnoff WD, Long KE, Kazura JW, Medof ME. 1992. Effect of glycoinositolphospholipid anchor lipid groups on functional properties of decay-accelerating factor protein in cells. J Biol Chem 261:356–362.
- Wojciechowicz D, Lu CF, Kurjan J, Lipke PN. 1993. Cell surface anchorage and ligand-binding domains of the Saccharomyces cerevisiae cell adhesion α-agglutinin, a member of the immunoglobulin superfamily. Mol Cell Biol 13:2554–2563.
- Xie FY, Woodle MC, Lu PY. 2006. Harnessing in vivo siRNA delivery for drug discovery and therapeutic development. Drug Discovery Today 11:67–73.
- Yu J, Fischman DA, Steck TL. 1973. Selective solubilization of proteins and phospholipids from red blood cell membranes by nonionic detergents. J Supramol Struct 1:233–243.
- Zechner R, Strauss JG, Haemmerle G, Lass A, Zimmermann R. 2005. Lipolysis: Pathway under construction. Curr Opin Lipidol 16:333–340.
- Zhang F, Schmidt WG, Hou Y, Williams AF, Jacobson K. 1992. Spontaneous incorporation of the glycosyl-phosphatidylinositol-linked protein Thy-1 into cell membranes. Proc Natl Acad Sci USA 89:5231–5235.