Abstract
The organ content of the mevalonate pathway lipids was investigated in liver-X-receptor (LXR) α, β and double knock-out mice. An extensive or moderate increase of total cholesterol in the double KO mice was found in all organs elicited by the increase of the esterified form. In LXRα and double KO mice, coenzyme Q (CoQ) was decreased in liver and increased in spleen, thymus and lung, while dolichol was increased in all organs investigated. This effect was confirmed using LXR- agonist GW 3965. Analysis of CoQ distribution in organelles showed that the modifications are present in all cellular compartments and that the increase of the lipid in mitochondria was the result of a net increase of CoQ without changing the number of mitochondria. It appears that LXR influences not only cellular cholesterol homeostasis but also the metabolism of CoQ and dolichol, in an indirect manner.
Introduction
The mevalonate pathway supplies farnesyl pyrophosphate as a common substrate for the synthesis of cholesterol, dolichol and coenzyme Q (CoQ) as well as for isoprenylation of a number of proteins (Goldstein and Brown Citation1990). In spite of the common initial biosynthetic pathway, these lipids have individual terminal regulation and fulfill different metabolic and functional requirements in the organism (Grunler et al. Citation1994). Cholesterol is a membrane component, substrate for the synthesis of bile acids and hormones and its dietary uptake is one-third of the amount utilized in the various tissues. Cholesterol is transported through the circulation and plasma levels of this lipid are of great medical importance. Dolichol is present in all tissues and membranes, with quantities increasing during aging; however, the function of the free alcohol has not yet been clarified. In contrast, dolichyl phosphate is an obligatory component in N-glycosylation of proteins, a process in which cytoplasmic water-soluble nucleotide activated sugars are transferred through the membranes to the site of glycosylation (Kornfeld and Kornfeld Citation1985).
Knowledge of the biological role of CoQ has greatly increased in recent years. This lipid is not only a member of the mitochondrial respiratory chain but also the only endogenously synthesized lipid-soluble antioxidant in humans (Turunen et al. Citation2004, Bentinger et al. Citation2007). CoQ also regulates the mitochondrial permeability transition pores, activates mitochondrial uncoupling proteins and is an important factor in blood immuno-regulatory processes (Echtay et al. Citation2001, Papucci et al. Citation2003, Schmelzer et al. Citation2007). In some genetic diseases, cardiomyopaties, degenerative muscle diseases and during aging, the CoQ tissue content decreases (Folkers et al. Citation1985, Kalen et al. Citation1989, Quinzii et al. Citation2008). In diseases dominated by oxidative stress such as diabetes, Alzheimer's and prion diseases the concentration is elevated (Soderberg et al. Citation1992, Guan et al. Citation1996).
Nuclear receptors regulate metabolic processes by controlling gene expression and it is expected that all mevalonate pathway lipids are subject to this type of regulation. Hepatocyte-specific retinoid-X-receptor-α- (RXRα) deficient mice exhibit decreased CoQ synthesis and content while cholesterol and dolichol are not affected (Bentinger et al. 2003b). Since the dimerization partner of the receptor involved in this process is not known, the mechanisms regulating CoQ synthesis are unclear. RXRα appears to be required for the basal transcription of genes involved in CoQ biosynthesis and also for its induction due to cold exposure. Depletion of the peroxisom proliferator-activated-receptor-α (PPARα) gene does not lead to any alterations in the lipid pattern of cholesterol, dolichol and CoQ in the liver (Turunen et al. Citation2000). PPARα is not required for the basal synthesis of these lipids; however, it is a necessary for the changes elicited by peroxisomal inducers. Treatment of rodents with peroxisomal inducers such as di(ethylhexyl)phthalate, clofibrate or salicylic acid induces the synthesis and content of CoQ in all organs with the exception of the brain, while cholesterol and dolichol levels remain unchanged (Aberg et al. Citation1994). In PPARα-null mice these inducers have no effect on the CoQ levels.
The liver-X-receptor (LXR) is a possible candidate for a regulatory role in CoQ biosynthesis. LXRα is widely distributed in metabolically active tissues, while the distribution of LXRβ is more restricted with the highest concentration being found in developing brain (Fan et al. Citation2008). LXRs function as main regulators of cholesterol homeostasis. They act as cholesterol sensors and regulate gene transcription upon activation with endogenous oxysterols (Zhao and Dahlman-Wright Citation2010). By activating bile acid synthesis and by increasing bile acid glucuronidation, cholesterol elimination from the body is efficient (Song et al. Citation2000, Barbier et al. Citation2009). LXRs stimulate reversed cholesterol transport, which involves transfer of accumulated cholesterol from peripheral tissues to the liver followed by biliary excretion (Naik et al. Citation2006). An additional function of LXRs is down-regulation of some of the cholesterol biosynthetic genes thereby causing suppression of cholesterol synthesis (Wang et al. Citation2008). It was also found that the low density lipoprotein receptor mediated uptake of cholesterol is influenced by LXRs. These nuclear receptors counteract cholesterol uptake from the intestine by reducing the activity of the transport system and at the same time activating the process of cholesterol loss by direct fecal excretion (Altmann et al. Citation2004, Duval et al. Citation2006). Additionally, LXRs have a role in fatty acid metabolism, as metabolic regulators of insulin action in the liver and lipogenesis in adipose tissue (Bensinger et al. Citation2008, Calkin and Tontonoz Citation2010). The broad impact of LXRs on cellular metabolism led to the interest to develop agonists as new therapeutic agents, which are now being tested in a number of in vitro and in vivo systems (Watanabe et al. Citation2005, Kratzer et al. Citation2009).
The initial biosynthetic sequence is common for all of the mevalonate pathway lipids and the terminal reactions of the individual lipids, cholesterol, dolichol and CoQ utilizes the same initial substrate, farnesyl-pyrophosphate. In spite of differences in terminal regulation of the individual end-products, the biosynthesis of these lipids is to some extent interdependent (Turunen et al. Citation2004). In this way LXR may influence not only cholesterol but also dolichol and CoQ synthesis.
In this study we analyzed the distribution of the mevalonate pathway lipids in different organs of LXRα-, β- and double knock-out mice and examined the effect of LXR agonist treatment. The results indicate that LXRα does not regulate but does influence the biosynthesis of dolichol and CoQ in an indirect manner.
Materials and methods
LXR knock-out mice
Two months old female WT and LXR knockout mice (Alberti et al. Citation2001) were housed on a regular 12 h light/12 h dark cycle with free access to water and food. All the experiments were approved by the local Ethical Committee on Animal Research in Northern and Southern Stockholm.
Mice were treated with LXR agonist GW 3965 perorally. The basal diet contained 18.5% protein, 55.7% carbohydrates and 4% fat. It did not contain cholesterol. Pellets were prepared containing 0.025% (w/w) of agonist. Food containing the LXR agonist was supplied ad libitum during 21 days.
Subcellular fractionation
For isolation of mitochondrial and lysosomal fractions a metrizamide gradient was used (Bentinger et al. 2003a). The 300 g supernatant of liver homogenate was centrifuged at 2800 g for 20 min to sediment mitochondria. The pellet was washed once by recentrifugation. This mitochondrial preparation (crude mitochondria) from 3 g tissue was resuspended in 10 ml 57% metrizamide, pH7.3, and placed in a SW 28 (Beckman, Fullerton, CA, USA) centrifuge tube. Above this fraction 6 ml 33%, 6 ml 26%, 6 ml 24.5%, and 7 ml 20% metrizamide solutions were layered. After centrifugation at 100,000 g for 3 h in the swinging bucket rotor, the fractions on top of every layer were removed with a syringe and numbered from the top to the bottom (1–5). In order to establish the type of organelles present in the various fractions, marker enzymes activities were measured. Fraction 1 was lysosomes and fractions 4 and 5 were mitochondria.
The supernatant after preparation of crude mitochondria was centrifuged at 10,000 g for 15 min and the supernatant obtained were centrifuged at 105,000 g for 60 min. The pellet made up the microsomal fraction.
In the case of spleen, the 300 g supernatant of the homogenate was layered over 5 ml of 26% metrizamide solution and centrifuged in a SW 41 rotor at 113,000 g for 3 h. The fraction on top of the metrizamide layer contained all the membraneous components of the cytosol, except for mitochondria, while the fraction on the bottom was the pure mitochondrial fraction.
Extraction and chromatography of lipids
The tissues were homogenized and the lipids were extracted from cells with chloroform:methanol:water (1:1:0.3), at 37°C for 1 h with magnetic stirring (Ericsson et al. Citation1992). The extracts thus obtained were adjusted to achieve a final chloroform:methanol:water ratio of 3:2:1 and complete phase separation was then accomplished by centrifugation. The lower chloroform phase was removed and evaporated to dryness under a flow of nitrogen and the residue subsequently re-dissolved in chloroform. These solutions were placed onto a silica column (50 mg/1.5 ml; Extract-Clean, Alltech, Deerfield, IL, USA) and thereafter eluted with 6 ml chloroform. After evaporation of the solvents, the neutral lipids were dissolved in chloroform:methanol (2:1).
For analysis of cholesterol, CoQ and dolichol, the samples were subjected to reversed-phase HPLC using a Supelcosil LC-18 column (3 μm, 4.0 × 75 mm) equipped with an LC-18 Supelguard (Supelco) column. For separation, a stepwise gradient involving methanol:water (9:1) in pump system A and methanol:2-propanol:hexane (2:1:1) in pump system B was employed at a flow rate of 1.1 ml/min. The gradient began at 15% solvent B, with increases to 47% after 8 min, 67% after 16 min and 100% after 24 min. The lipids in the eluent were monitored at 210 and 275 nm with UV detector. Ergosterol, CoQ6 and dolichol-23 were used as internal standards.
In order to determine the portion of cholesterol and dolichol that is esterified, the neutral lipid extract was subjected to alkaline hydrolysis. After evaporation of the solvent, the lipids were dissolved in 0.5 ml benzene and 0.5 ml 15% KOH in 95% ethanol was added. After incubation at 95°C for 60 min the mixture was mixed with 0.5 ml water and 1 ml hexane to obtain a phase separation. The upper hexane phase was washed with a mixture of 0.5 ml 150 mM NaCl and 0.1 ml ethanol. HPLC analyses were performed as described above. In this procedure the cholesterol fraction represents both the free and esterified forms which are also valid for the dolichol fraction.
Malondialdehyde
The tissues were homogenized (1 g per 15 ml 150 mM NaCl) containing 1 mM desferroxamin, 1 mM EDTA, 1 mM EGTA and 2 mM butylated hydroxytoluene. 95 μl of the homogenate was mixed with 5 μl PCA (70–72%). After 10 min the mixture was centrifuged at 13,000 rpm for 10 min. 70 μl of the supernatant were mixed with 8 μl 7.5 mM dinitrophenyhydrazine, dissolved in 2 M HCl. This mixture was kept at room temperature 30 min, protected from light. 26 μl was injected into HPLC, using a reversed phase Supelco C18 T column. Separation was performed by using a gradient with acetonitril:water (1:2) in pump system A and acetonitril:water (5:1) in pump system B at a flow rate of 1 ml/min. The gradient started with 100% solvent A reaching 60% solvent B after 10 min and 100% after 12 min. The eluate was monitored at 307 nm.
Cytochrome oxidase and protein
Cytochrome oxidase was measured by following the oxidation of reduced cytochrome c at 550 nm (Forsmark-Andree et al. Citation1997). Protein concentrations were measured using the Bicinchoninic acid kit for protein determination (Sigma).
Statistical analysis
The results obtained are expressed as means ± SD and the differences between groups were evaluated for statistical significance by analyzes with ANOVA, followed by Dunnett's test. P values below 0.05 were considered statistically significant.
Results
Mevalonate pathway lipids and MDA in liver and spleen
In these studies the lipids extracted from tissues were subjected to silica chromatography in order to remove phospholipids and then analyzed with and without alkaline hydrolysis. This latter step is required since a substantial portion of both cholesterol and dolichol can be present in esterified form both in control and LXR-KO mice. The cholesterol content in the liver is doubled in the LXR double KO mice, mainly due to an increase in the esterified forms (). In this organ about one-third of the dolichol is esterified, and the three different types of LXR-KO mice do not show any variations in concentration. In contrast, the content of CoQ is significantly decreased in LXR alpha and double KO mice but not in beta KO mice. Since one of the main functions of CoQ is to serve as a lipid-soluble antioxidant, it is possible that the differences in concentration of this lipid represent a response to the oxidative stress conditions in the organ. In fact, the breakdown product of lipid peroxidation, MDA, is decreased in the livers of both LXR alpha and double KO mice. Similarly to liver, spleen of double KO mice also exhibits a large increase in esterified cholesterol (). Dolichol and CoQ follow the same pattern, showing increased amounts in LXR alpha and double KO mice. The increase in MDA is parallel to the increase in CoQ only in the case of the double KO. The beta KO, but not the alpha KO mice, showed an increased MDA amount.
Figure 1. Mevalonate pathway lipids and malondialdehyde in liver (A) and spleen (B) of LXRα, -β and double KO mice. The columns in the case of cholesterol and dolichol give both the free and the esterified forms. The values are means ± SD (n = 9). This Figure is reproduced in color in the online version of Molecular Membrane Biology.
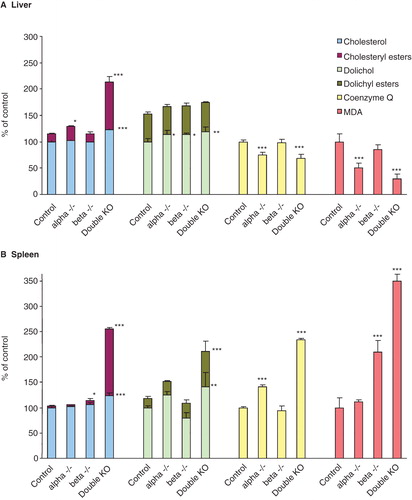
A change in lipid composition was also found to some extent in other organs. The observation in thymus is similar to that seen in spleen with the exception of dolichol which decreased in the beta KO and the double KO mice (). Esterified cholesterol is greatly increased in the LXR double KO mice, while CoQ is increased in all types of KO mice. The kidney differs somewhat from the other organs as the LXR beta KO mice display the highest CoQ amount (). In heart the changes are restricted to an increase in esterified dolichol at expense of free dolichol (). The picture in the lung follows that of the spleen and the thymus, an increase in esterified cholesterol in LXR double KO mice and an elevation of dolichol and CoQ content in LXR alpha and double KO mice ().
Figure 2. Mevalonate pathway lipids in thymus (A), kidney (B), heart (C) and lung (D) of LXRα, -β and double KO mice. The columns in the case of cholesterol and dolichol give both the free and the esterified forms. The values are means ± SD (n = 9). This Figure is reproduced in color in the online version of Molecular Membrane Biology.
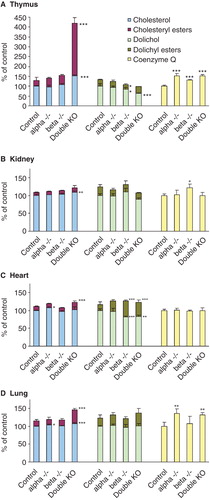
Figure 3. Effects of LXR agonist GW 3965 on the concentrations of mevalonate pathway lipids in liver (A) and spleen (B). In spleen the amount of cholesteryl esters are not given since these values are very low and at the sensitivity limit for detection. The values are means ± SD (n = 4). This Figure is reproduced in color in the online version of Molecular Membrane Biology.
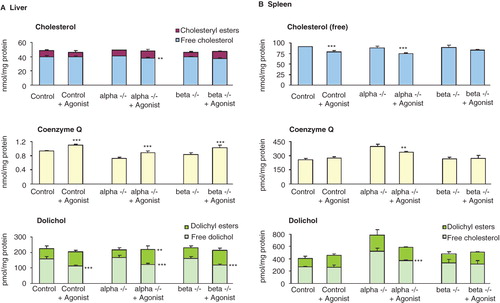
LXR agonist treatment
In order to further investigate the effect of LXR on the mevalonate pathway lipids, the mice were treated with a LXR agonist. In liver, the amount of cholesterol is not modified to any larger extent upon treatment (). The CoQ amount is decreased in the liver, both in alpha and double KO mice, while upon treatment with agonist an increased amount were found not only in the control liver but also in the alpha and beta KO mice. Treatment with LXR agonist did not change the total dolichol amount; however, the esterified portions were increased.
The agonist treatment also affected the lipid composition in the spleen (). In the case of free cholesterol there is tendency of decrease in all mice. The treatment resulted in a lowered concentration of CoQ and total dolichol in alpha but not in beta KO mice.
Subcellular distribution of mevalonate pathway lipids
Lipids are specifically involved in structural and metabolic processes and therefore it is of importance to know the subcellular distribution after a change in the total cellular concentration. Total liver homogenate of the LXR double KO mice exhibited an increase in free cholesterol and dolichol and a decrease in CoQ content in all subcellular organelles (). The distribution of lipids is substantially different in the various organelles, and in addition, the number of organelles is also very different in the cell, e.g., lysosomes represent only a small portion of the cellular protein in comparison with mitochondria and microsomes. The cholesterol elevation was mainly due to the lysosomal and microsomal contribution and these fractions had also the major decrease in CoQ. On the other hand, the increase in dolichol was most prominent in lysosomes, however, on a protein level the microsomal and mitochondrial fractions significantly contributed to the modifications since these fractions are the two major components of the liver cell.
Table I. Subcellular distribution of mevalonate pathway lipids in liver.
The spleen was also investigated concerning intracellular lipid distribution of the three mevalonate pathway lipids, cholesterol, CoQ and dolichol (). The small amount of tissue available does not allow a complete fractionation and for this reason a Metrizamide gradient was used to isolate mitochondria not contaminated with lysosomes. The starting material was nuclear free homogenate and all the remaining organelles in the upper part of the gradient was centrifuged down into one fraction, called ‘other organelles' containing all cytoplasmic components with the exception of nucleus and mitochondria. The amount of cholesterol, CoQ and dolichol is moderately increased in LXR alpha KO and more extensively in double KO mice. The increase in amount of mevalonate pathway lipids in mitochondria and other organelles were very similar, indicating a general influence of LXR on lipid metabolism concerning the mevalonate pathway, leading to an even cellular distribution of the newly synthesized components.
Table II. Subcellular distribution of mevalonate pathway lipids in spleen.
Mitochondrial CoQ
The amount of mitochondrial protein isolated from liver and spleen did not show any differences between control and KO mice, indicating that the increase or decrease in CoQ amount is not related to changes in the mitochondrial number. Cytochrome oxidase is the terminal enzyme in the mitochondrial respiratory chain and its concentration and activity is known to be proportional to the amount of mitochondria under various physiological conditions. Consequently, its activity is a measure of the mitochondrial amount in the homogenate. When calculating the CoQ amount in liver in relation to cytochrome oxidase activity, the amount in the LXR alpha and double KO mice is lower than in the control (). In the spleen the situation is the opposite, the calculated CoQ amount is higher in these two knock-out mice (). These results demonstrate that modifications in CoQ amount are not elicited by changes in the number of mitochondria but by changes in the CoQ concentration in the individual organelles.
Discussion
A number of regulatory mechanisms, including receptors, intermediates and modified metabolites are described for cholesterol synthesis which is of considerable biological and medical interest. Various types of drugs for treatment of hypercholesterolemia have been developed by employing the information obtained from these studies (Schaefer Citation2011). In contrast to cholesterol, there are no regulatory mechanisms known for CoQ and consequently, no drugs are available to regulate its biosynthesis.
Low level of tissue CoQ is a common defect and characteristic for several conditions. During aging both in rodents and human, tissue levels of the lipid are continuously decreasing (Kalen et al. Citation1989). The common condition of cardiomyopathy is associated with low heart concentration of CoQ which is considered to be a contributing factor for deficient mitochondrial respiration and energy production (Littarru and Tiano Citation2010). Development of experimental and human hepatocarcinoma also results in decreased CoQ levels (Eggens et al. Citation1989). On the other hand, conditions that are characterized by increased oxidative stress, such as diabetes, Alzheimer's and prion diseases respond with elevated levels of CoQ (Soderberg et al. Citation1992, Guan et al. Citation1996). These events demonstrate that regulatory mechanisms are operating to activate or depress CoQ synthesis in various metabolic states. In the case of cholesterol both nuclear receptors and endogenous metabolites such as oxysterols, squalene dioxide, farnesol and its derivatives, geranylgeraniol, prenyl phosphates and sterols that activate meiosis exert regulatory functions (Edwards and Ericsson Citation1999). In the case of CoQ, regulatory mechanisms are not yet established but the situation should be similar to that of cholesterol. A receptor having RXRα as dimeric partner appears to be involved in the regulation of the synthesis of this lipid. In addition, in rodent but not in human, PPARα is required for upregulation of the biosynthetic system upon employing peroxisomal inducers (Aberg et al. Citation1994). Metabolic intermediates affecting CoQ synthesis are not yet studied in detail but it appears that epoxidated all-trans polyisoprenols are efficient in upregulating CoQ synthesis (Bentinger et al. Citation2008).
LXRs influence CoQ synthesis without directly regulating the process. The most prominent modifications in amount are found in the double KO mice, apparently elicited mainly by LXRα. Since the changes are organ specific, they may be caused by different metabolic responses mediated by LXRα. CoQ amount is decreased in liver and increased in spleen, thymus and lung which may be a result of a decrease or an increase in oxidative stress. Malondialdehyde, a product of lipid peroxidation, is decreased in liver and increased in spleen of LXRα-KO mice where as the main lipid soluble endogenous antioxidant, CoQ is simultaneously decreased and increased, respectively in these two organs. In pathological conditions where oxidative stress plays a significant role, cellular CoQ synthesis is increased in an effort to create elevated antioxidant protection (Bentinger et al. Citation2010). It appears that this elevation is not sufficient to quench the free radicals produced in the spleen where the increase of CoQ was also paralleled by an increase of malondialdehyde. It is known that in conditions where oxidative stress play a major role, such as diabetes, Alzheimer's and prion diseases, an increase of the CoQ content takes place in some tissues (Soderberg et al. Citation1992, Guan et al. Citation1996).
The validity of the involvement of LXRα in modulation of tissue CoQ concentration is further proven by treatment of KO mice with the agonist GW 3965. The treatment affected both the control and the LXRα-KO mice and either increased or decreased the CoQ amount in different organs. The general effect of the LXR-agonist was that the changes in lipid amount (seen in the KO-mice) were normalized, almost reaching the values of the control mice.
Cellular changes are often elicited by modification of cellular organization and alteration in the amount and structure of the subcellular organelles. A number of drugs cause proliferation of the endoplasmic reticulum with great enrichment of the cytochrome P-450 detoxification system in these membranes (Ernster and Orrenius Citation1973). Inducers of PPARα such as diethylhexylphthalate, perflurooctanoic acid and salicylic acid selectively affect peroxisomes and increase several-fold peroxisomal fatty acid β-oxidation (van den Bosch et al. Citation1992). CoQ changes in mice by knockout of the LXRα or an agonist of it, lead on the other hand to a more extended effect on the cellular structure and lipid modifications are not restricted to a single compartment but influence all organelles. This fact could be proven by analyses of isolated subcellular fractions both in liver and spleen. Concerning CoQ, an interesting finding is that increase or decrease in the amount of the lipid is not paralleled by changes in the number of mitochondria. Accordingly, the quantitative composition of the lipids in the mitochondrial inner membrane can be modified, which means a considerable structural reorganization of the membrane structure.
Cholesterol, the main product of the mevalonate pathway, exhibits different behavior than the other lipids of the pathway. In all organs there is a moderate or extensive increase of total cholesterol in the double KO mice. The elevation is elicited by the esterified form while free cholesterol is more or less unchanged. The accumulation of cholesterol agrees with the established role of LXR in cholesterol transport.
Dolichol is present in all cells and tissues and is one of the three main products of the mevalonate pathway. Its phosphorylated form is a crucial carrier in glycoprotein synthesis but the major part is present as free alcohol with an as not yet established function (Swiezewska and Danikiewicz Citation2005). A part of this polyprenol is esterified with a fatty acid in all tissues. In most organs of LXRα and LXR double KO mice, but not in liver, the distribution follows that of CoQ, with a minor or more extensive increase. As with cholesterol, the esterified form increases preferentially (Tollbom et al. Citation1989). The esterification process appears to be the main target, an observation which was confirmed by the agonist treatment of both control and KO mice, since the esterified part of dolichol is the fraction which is mainly increased.
A large number of important cellular functions are influenced and regulated by the mevalonate pathway lipids and modifications in their amounts and metabolism are established in a number of physiological and pathophysiological conditions. In a non-direct way LXR seems to interfere not only with the cellular cholesterol homeostasis but also with the metabolism of CoQ which is of importance since this lipid is a general component of endomembranes and participate in several basic functions required for normal life.
Conclusion
LXR plays a key role in cholesterol homeostasis which raised the possibility that this nuclear receptor also has a regulatory function for CoQ synthesis. This assumption is based on the previous finding that partial deletion of RXRα greatly decreased CoQ synthesis in the liver which suggested that LXR may be a candidate for the heterodimeric receptor that regulates the synthesis of this lipid. Cholesterol, mainly in the esterified form, is to various extents increased in all organs of double KO mice, while the CoQ amount in LXRα and double KO mice is decreased in liver, significantly increased in spleen, thymus and lung but remained unchanged in kidney and heart. An increased concentration of dolichol in the LXRα and double KO mice was also observed in several organs. Treatment of LXRα mice with the agonist GW 3965 showed the opposite change in the lipid amount, almost normalizing the values. Analyses of the cellular organelles show that no major changes in the organelle distribution occurs as a consequence of LXR deficiency and the lipid changes are restricted to the existing organelles. The results show that LXR does not directly regulate CoQ and dolichol synthesis but influences the metabolism of these lipids in an indirect way.
Acknowledgements
This work is supported by the Swedish Research Council and the Family Erling-Persson Foundation.
Declaration of interest: The authors report no conflicts of interest. The authors alone are responsible for the content and writing of the paper.
References
- Aberg F, Zhang Y, Appelkvist EL, Dallner G. 1994. Effects of clofibrate, phthalates and probucol on ubiquinone levels. Chem Biol Interact 91:1–14.
- Alberti S, Schuster G, Parini P, Feltkamp D, Diczfalusy U, Rudling M, 2001. Hepatic cholesterol metabolism and resistance to dietary cholesterol in LXRbeta-deficient mice. J Clin Invest 107:565–573.
- Altmann SW, Davis HR Jr, Zhu LJ, Yao X, Hoos LM, Tetzloff G, 2004. Niemann-Pick C1 Like 1 protein is critical for intestinal cholesterol absorption. Science 303:1201–1204.
- Barbier O, Trottier J, Kaeding J, Caron P, Verreault M. 2009. Lipid-activated transcription factors control bile acid glucuronidation. Mol Cell Biochem 326:3–8.
- Bensinger SJ, Bradley MN, Joseph SB, Zelcer N, Janssen EM, Hausner MA, 2008. LXR signaling couples sterol metabolism to proliferation in the acquired immune response. Cell 134:97–111.
- Bentinger M, Brismar K, Dallner G. 2007. The antioxidant role of coenzyme Q. Mitochondrion 7(Suppl):S41–S50.
- Bentinger M, Dallner G, Chojnacki T, Swiezewska E. 2003a. Distribution and breakdown of labeled coenzyme Q10 in rat. Free Radic Biol Med 34:563–575.
- Bentinger M, Tekle M, Brismar K, Chojnacki T, Swiezewska E, Dallner G. 2008. Polyisoprenoid epoxides stimulate the biosynthesis of coenzyme Q and inhibit cholesterol synthesis. J Biol Chem 283:14645–14653.
- Bentinger M, Tekle M, Dallner G. 2010. Coenzyme Q – biosynthesis and functions. Biochem Biophys Res Commun 396:74–79.
- Bentinger M, Turunen M, Zhang XX, Wan YJ, Dallner G. 2003b. Involvement of retinoid X receptor alpha in coenzyme Q metabolism. J Mol Biol 326:795–803.
- Calkin AC, Tontonoz P. 2010. Liver x receptor signaling pathways and atherosclerosis. Arterioscler Thromb Vasc Biol 30:1513–1518.
- Duval C, Touche V, Tailleux A, Fruchart JC, Fievet C, Clavey V, 2006. Niemann-Pick C1 like 1 gene expression is down-regulated by LXR activators in the intestine. Biochem Biophys Res Commun 340:1259–1263.
- Echtay KS, Winkler E, Frischmuth K, Klingenberg M. 2001. Uncoupling proteins 2 and 3 are highly active H(+) transporters and highly nucleotide sensitive when activated by coenzyme Q (ubiquinone). Proc Natl Acad Sci USA 98:1416–1421.
- Edwards PA, Ericsson J. 1999. Sterols and isoprenoids: Signaling molecules derived from the cholesterol biosynthetic pathway. Annu Rev Biochem 68:157–185.
- Eggens I, Elmberger PG, Low P. 1989. Polyisoprenoid, cholesterol and ubiquinone levels in human hepatocellular carcinomas. Br J Exp Pathol 70:83–92.
- Ericsson J, Appelkvist EL, Thelin A, Chojnacki T, Dallner G. 1992. Isoprenoid biosynthesis in rat liver peroxisomes. Characterization of cis-prenyltransferase and squalene synthetase. J Biol Chem 267:18708–18714.
- Ernster L, Orrenius S. 1973. Dynamic organization of endoplasmic reticulum membranes. Drug Metab Dispos 1:66–73.
- Fan X, Kim HJ, Bouton D, Warner M, Gustafsson JA. 2008. Expression of liver X receptor beta is essential for formation of superficial cortical layers and migration of later-born neurons. Proc Natl Acad Sci USA 105:13445–13450.
- Folkers K, Vadhanavikit S, Mortensen SA. 1985. Biochemical rationale and myocardial tissue data on the effective therapy of cardiomyopathy with coenzyme Q10. Proc Natl Acad Sci USA 82:901–904.
- Forsmark-Andree P, Lee CP, Dallner G, Ernster L. 1997. Lipid peroxidation and changes in the ubiquinone content and the respiratory chain enzymes of submitochondrial particles. Free Radic Biol Med 22:391–400.
- Goldstein JL, Brown MS. 1990. Regulation of the mevalonate pathway. Nature 343:425–430.
- Grunler J, Ericsson J, Dallner G. 1994. Branch-point reactions in the biosynthesis of cholesterol, dolichol, ubiquinone and prenylated proteins. Biochim Biophys Acta 1212:259–277.
- Guan Z, Soderberg M, Sindelar P, Prusiner SB, Kristensson K, Dallner G. 1996. Lipid composition in scrapie-infected mouse brain: Prion infection increases the levels of dolichyl phosphate and ubiquinone. J Neurochem 66:277–285.
- Kalen A, Appelkvist EL, Dallner G. 1989. Age-related changes in the lipid compositions of rat and human tissues. Lipids 24:579–584.
- Kornfeld R, Kornfeld S. 1985. Assembly of asparagine-linked oligosaccharides. Annu Rev Biochem 54:631–664.
- Kratzer A, Buchebner M, Pfeifer T, Becker TM, Uray G, Miyazaki M, 2009. Synthetic LXR agonist attenuates plaque formation in apoE-/- mice without inducing liver steatosis and hypertriglyceridemia. J Lipid Res 50:312–326.
- Littarru GP, Tiano L. 2010. Clinical aspects of coenzyme Q10: An update. Nutrition 26:250–254.
- Naik SU, Wang X, Da Silva JS, Jaye M, Macphee CH, Reilly MP, 2006. Pharmacological activation of liver X receptors promotes reverse cholesterol transport in vivo. Circulation 113:90–97.
- Papucci L, Schiavone N, Witort E, Donnini M, Lapucci A, Tempestini A, 2003. Coenzyme q10 prevents apoptosis by inhibiting mitochondrial depolarization independently of its free radical scavenging property. J Biol Chem 278:28220–28228.
- Quinzii CM, Lopez LC, Naini A, Dimauro S, Hirano M. 2008. Human CoQ10 deficiencies. Biofactors 32:113–118.
- Schaefer JR. 2011. Lipid management for the prevention of cardiovascular disease. Curr Pharm Des 17:852–860.
- Schmelzer C, Lindner I, Vock C, Fujii K, Doring F. 2007. Functional connections and pathways of coenzyme Q10-inducible genes: An in-silico study. IUBMB Life 59:628–633.
- Soderberg M, Edlund C, Alafuzoff I, Kristensson K, Dallner G. 1992. Lipid composition in different regions of the brain in Alzheimer's disease/senile dementia of Alzheimer's type. J Neurochem 59:1646–16453.
- Song C, Hiipakka RA, Liao S. 2000. Selective activation of liver X receptor alpha by 6alpha-hydroxy bile acids and analogs. Steroids 65:423–427.
- Swiezewska E, Danikiewicz W. 2005. Polyisoprenoids: Structure, biosynthesis and function. Prog Lipid Res 44:235–258.
- Tollbom O, Chojnacki T, Dallner G. 1989. Hydrolysis of dolichyl esters by rat liver lysosomes. J Biol Chem 264:9836–9841.
- Turunen M, Olsson J, Dallner G. 2004. Metabolism and function of coenzyme Q. Biochim Biophys Acta 1660:171–199.
- Turunen M, Peters JM, Gonzalez FJ, Schedin S, Dallner G. 2000. Influence of peroxisome proliferator-activated receptor alpha on ubiquinone biosynthesis. J Mol Biol 297:607–614.
- Van Den Bosch H, Schutgens RB, Wanders RJ, Tager JM. 1992. Biochemistry of peroxisomes. Annu Rev Biochem 61:157–197.
- Wang Y, Rogers PM, Su C, Varga G, Stayrook KR, Burris TP. 2008. Regulation of cholesterologenesis by the oxysterol receptor, LXRalpha. J Biol Chem 283:26332–26339.
- Watanabe Y, Jiang S, Takabe W, Ohashi R, Tanaka T, Uchiyama Y, 2005. Expression of the LXRalpha protein in human atherosclerotic lesions. Arterioscler Thromb Vasc Biol 25:622–627.
- Zhao C, Dahlman-Wright K. 2010. Liver X receptor in cholesterol metabolism. J Endocrinol 204:233–240.