Abstract
Membrane-bound pyrophosphatases (M-PPases) are enzymes that enhance the survival of plants, protozoans and prokaryotes in energy constraining stress conditions. These proteins use pyrophosphate, a waste product of cellular metabolism, as an energy source for sodium or proton pumping. To study the structure and function of these enzymes we have crystallized two membrane-bound pyrophosphatases recombinantly produced in Saccharomyces cerevisae: the sodium pumping enzyme of Thermotoga maritima (TmPPase) and the proton pumping enzyme of Pyrobaculum aerophilum (PaPPase). Extensive crystal optimization has allowed us to grow crystals of TmPPase that diffract to a resolution of 2.6 Å. The decisive step in this optimization was in-column detergent exchange during the two-step purification procedure. Dodecyl maltoside was used for high temperature solubilization of TmPPase and then exchanged to a series of different detergents. After extensive screening, the new detergent, octyl glucose neopentyl glycol, was found to be the optimal for TmPPase but not PaPPase.
Introduction
Membrane-bound pyrophosphatases (M-PPases, EC 3.6.1.1) are homodimeric (Kellosalo et al. Citation2011) integral membrane proteins that catalyze a reaction where the energy released from pyrophosphate hydrolysis is converted to ion pumping. On the basis of their activity M-PPases can be divided into three functional classes: K+-dependent sodium pumps, K+-dependent proton pumps and K+-independent proton pumps (Malinen et al. Citation2007).
M-PPases are found in plants, protozoans and many prokaryotes (Serrano et al. Citation2007) and enhance the survival of these organisms in conditions like low light intensity, anoxia, salt stress and cold, where cells face stress due to limited energy (Garcia-Contreras et al. Citation2004, Lopéz-Marqués et al. Citation2004, Serrano et al. Citation2007). M-PPases are also important for maturation of plants (Maeshima Citation2000). Inhibitors of M-PPases, such as bisphosphonates and imidodiphosphates, reduce the proliferation of disease-causing protozoans like Trypanosoma cruzi (McIntosh et al. Citation2001, Lemercier et al. Citation2002). Also, knock-out of Trypanosoma brucei M-PPase expression through RNA interference leads to reduced growth and induces loss of functional acidocalsisomes (Lemercier et al. Citation2002). For these reasons M-PPases represent a possible drug target for treating diseases like malaria, leishmaniasis and African sleeping sickness caused by protozoan organisms (McIntosh and Vaidya Citation2002). This is especially true as humans and other mammals do not have an M-PPase.
The highly hydrophobic M-PPases contain 14–17 transmembrane helices (Mimura et al. Citation2004), with conserved motifs in the cytoplasmic part of the protein (Maeshima Citation2000). These motifs are essential for the binding of Mg2+, which is essential for activity, and the substrate Mg2PPi (Maeshima Citation2000). There is no sequence or structural similarity between M-PPases and soluble pryophosphatases, nor between M-PPases and the other formally-similar pumps (the V-, F-, A- and P-type ATPases) that use high energy phosphoanhydride compounds. Consequently, understanding how M-PPases represent a unique solution to ion transportation and pyrophosphate hydrolysis is of structural, mechanistic and biomedical relevance.
In this paper we report the crystallization and preliminary X-ray analysis of a sodium pumping pyrophosphatase from Thermotoga maritima (TmPPase) and proton pumping pyrophosphatase of Pyrobaculum aerophilum (PaPPase). We also present the results of the optimization of TmPPase crystals, which have allowed us to grow crystals diffracting to 2.6 Å.
Materials and methods
Expression and purification of TmPPase and PaPPase
N-terminally His6-tagged TmPPase and PaPPase were expressed in BJ1991-strain Saccharomyces cerevisae cells under a constitutive PMA1-promoter (Kellosalo et al. Citation2011). The proteins were purified according to a modified version of ‘hot-solve' method (López-Marqués et al. Citation2005). The solubilization of PaPPase was carried with 0.67% dodecyl-β-maltopyranoside (DDM, 99% pure, Melford laboratories) while 1.33% DDM was used for the solubilization of TmPPase. In most of the purification batches the dodecyl-β-maltopyranoside was exchanged for another detergent during the washing and elution steps of Ni-affinity chromatography ().
Table I. Detergents used in detergent exchange of TmPPase and PaPPase.
Binding of the proteins to the Ni-affinity matrix was performed in batches at 40°C (López-Marqués et al. Citation2005). Washing and elution steps were carried out in a column with two column volumes of washing buffer (50 mM MES-NaOH pH 6.5, 20% glycerol, 50 mM KCl, 5 mM MgCl2, 1 mM Na2HPO4, 20 mM imidazole and 1 mM DTT) and two column volumes of elution buffer (50 mM MES-NaOH pH 6.5, 3.5% glycerol, 50 mM KCl, 5 mM MgCl2, 1 mM Na2HPO4, 400 mM imidazole and 1 mM DTT). In addition to the aforementioned components, the washing and elution buffers also contained detergent (). The pyrophosphatase activity of the purified proteins (TmPPase CYM-4, DMNPG and OGNPG and PaPPase OGNPG) was assayed as described previously (Kellosalo et al. Citation2011).
Crystallization of TmPPase and PaPPase
The purified TmPPase and PaPPase were concentrated using Amicon® Ultra 50,000 MWCO (Millipore) concentrators and buffer exchanged to crystallization buffer (20 mM MES-NaOH pH 6.5, 3.5% (v/v) glycerol, 50 mM KCl, 5 mM MgCl2, 1 mM Na2HPO4, 2 mM DTT and 0.05% DDM) with Micro Bio-Spin® 6 columns (Bio-Rad). If the detergent had been exchanged during Ni-affinity purification, the same detergent was used in the crystallization buffer in place of DDM at the concentration used during Ni-affinity chromatography (see above). The buffer exchanged protein was diluted to a concentration of 10 mg/ml with crystallization buffer for the subsequent crystallization trials.
To crystallize TmPPase and PaPPase in each detergent, we set up MemGold™ (Molecular Dimensions) and other sparse matrix screens up at 21°C and 4°C. These were carried out as sitting-drop vapour diffusion experiments with 0.2–0.4 μl drops (0.1–0.2 μl protein-solution + 0.1–0.2 μl well solution) with 80 μl reservoir volume and were set-up with Mosquito®LCP pipetting robot (TTP Labtech) or a Cartesian Microsys nanodispenser (Genomic solutions®) on Innovaplate™ SD-2-plates (Innovadyne). Duplicate drops were set up of each condition because we noticed early on that crystal formation was unpredictable. In order to obtain better diffracting crystals, hits from these screens were further optimized by first optimizing PEG and salt concentrations and pH and then carrying out the crystallizations with 1–2 μl drops (0.5–1 μl protein-solution + 0.5–1 μl well solution, done on Cryschem 24-well plates (Hampton Research) with 1 ml reservoir volume (). Optimization of hits of TmPPase in DDM, UDM, DM, CYM-6 and OGNPG and PaPPase in DDM and DM was also tested with Hampton Research's Detergent HT screen with 2–5 × CMC of additive detergents and Additive HT screen and with various secondary detergents. Crystallization of TmPPase in DDM was also tested in different PEGs (PEG 200, PEG 400, PEG 550 MME, PEG 1000, PEG 3350 and PEG 4000).
Table II. Crystallization of TmPPase and PaPPase in DDM.
Crystal optimization by addition of lipids was also tested using a modification of the ‘HiLiDe'- protocol (Gourdon et al. Citation2011). In these experiments 0.1, 0.5, 1 or 2 mg/ml of dioleyl phosphatidyl choline (DOPC, Avanti Polar lipids) was added to protein preparations containing either 10 mg/ml of TmPPase or PaPPase in 0.45% DM. Additional mixtures of preparations containing protein with 1 or 2 mg/ml of DOPC were made by adding 0.1% or 0.2% DM to them. In order to reduce evaporation and prepare the mixtures in as small volume as possible, the inert nitrogen gas used to overlay the samples was humidified by bubbling it through water. After overnight incubation and ultracentrifugation, MemGold screens were set up of the preparations at 21°C. These were done as sitting-drop vapour diffusion experiments with 0.4 μl drops (0.2 μl protein-solution + 0.2 μl well solution) with 80 μl reservoir volume and were set-up using the Mosquito®LCP pipetting robot in Innovaplate™ SD-2-plates.
X-ray diffraction experiments
The crystals harvested from 0.4 μl drops were frozen in the presence of mother liquor with liquid nitrogen. Crystals from 2 μl drops were first cryoprotected by transferring them to artificial mother liquor containing 25–30% PEG 350 MME, 0.2 M CaCl2, 0.1 M MES-NaOH pH 6.5, 3.5% glycerol, 50 mM KCl, 1 mM Na2HPO4, 2 mM DTT and 0.2% OGNPG. The frozen crystals were taken to the European Synchrotron Radiation Facility (ESRF, Grenoble, France), National Electron Accelerator Laboratory for Synchrotron Radiation Research, Nuclear Physics and Accelerator Physics (MAX-lab, Lund, Sweden) and Swiss Light Source (SLS, Villigen, Swizerland) for X-ray diffraction experiments. Experiments were carried out on beam-lines ID14-1, ID14-2, ID-14-4, BM-14, ID-23-1, ID-23-2 and ID-29 at ESRF, I911-3 at MAX-lab and X06SA at SLS. The diffraction experiment that gave the best results was carried out on beam line I911-3 with 5 s exposure and 100% transmission. For this crystal, a data-set was collected with 1° oscillation using a MarMosaic 225 detector. All the collected data were processed with XDS (Kabsch Citation1993).
Results
Activity measurements of purified proteins
As the effect of the detergents of the first and second round of detergent exchange on the activities of TmPPase and PaPPase has been tested previously (Kellosalo et al. Citation2011) we measured the pyrophosphate hydrolysis activities of TmPPase purified in OGNPG, DMNPG and CYM-4 and PaPPase purified in OGNPG. The activity of both of the proteins in these detergents is 80–100% of their activity in DDM ().
Optimization of crystals of TmPPase and PaPPase purified in DDM
TmPPase and PaPPase were heterologously expessed in Saccharomyces cerevisae and purified according to the ‘hot-solve' protocol (López-Marqués et al. Citation2005). Following the original protocol we initially purified both PaPPase and TmPPase in DDM. After buffer exchanging the proteins into a crystallization buffer containing all the ligands of M-PPases (K+, Na+, Mg2+ and PO4 2-), we set up crystallisation screens similar to that described by Iwata (Citation2003) at 21°C with 0.2 μl drops using the sitting-drop vapour diffusion method.
Crystals grew in different PEG 400 containing conditions of this screen. These small TmPPase and PaPPase crystals diffracted to 7.5 Å and 28 Å, respectively ().
We tried optimizing the crystallization conditions by testing different PEG-concentrations (20–40% PEG 400), salt concentration (0–0.7 M NaCl), pH (4–9), changing the precipitant (PEG 200, PEG 550 MME, PEG 1000, PEG 3350 and PEG 4000 instead of PEG 400), adding secondary detergents and additives (Hampton additive HT screen), lowering the DDM concentration in the Ni-affinity and crystallization buffers (from 0.08% to 0.05% or 0.02%), crystallizing at 4°C, and using bigger drops (0.5 μl protein solution + 0.5 μl well solution). Altogether we harvested over 50 crystals from different conditions and took these to various beamlines at the ESRF (see Methods) to test their diffraction.
One TmPPase crystal grown at 4°C diffracted to 6.5 Å. Use of nonyl glucoside and CHAPSO as secondary detergents also brought an improvement in resolution (). The diffraction from the secondary detergent containing TmPPase crystals was, however, severely anisotropic: the resolution of the diffraction was 4.5 Å in one direction, but only 8 Å in other. Too low DDM concentration seemed to hinder crystallization as no crystals grew from a TmPPase preparation in which the buffer solution contained only 0.02% DDM (≈2 × CMC) ().
The TmPPase in DDM crystallized in two or three crystal forms, with the crystals grown in the presence of Li2SO4, NaCl and Novelle buffer 4 (Newman Citation2004) forming crystals with the space group P212121 and crystals grown in the presence of NaCl and citrate forming crystals with the space group P422 (). The addition of NG or CHAPSO to the citrate and NaCl containing crystallization conditions caused the space group to change to primitive orthorhombic (). As the diffraction from these crystals was highly anisotropic we could not assign the space groups with lattice forms.
The optimization of the crystallization conditions of TmPPase and PaPPase purified in DDM was hampered by the irreproducibility of crystal growth between different protein batches and even identical drops on the same crystallization plate; the PEG concentration and pH in which the crystals appeared varied between 20 and 40% and 4–9, respectively. The diffraction quality of the crystals was also highly variable varying from worse than 20 Å to about 6 Å between crystals from identical set-ups (unpublished work, J.K & T.K.). Protein crystals were quite small (50 × 30 × 30 μm at their largest) even when 1 μl drops were employed. Due to these problems and due to the modest improvement in diffraction resolution we stopped working on crystals grown from DDM.
Optimization of TmPPase and PaPPase crystals by detergent exchange
We carried out detergent exchange on DDM solubilized TmPPase and PaPPase by having a different detergent in the wash and elution buffers of Ni-affinity chromatography and in the crystallization buffer (). We chose the detergents largely on the basis on previous purification trials (Kellosalo et al. Citation2011).
Initially we tested crystallization of TmPPase in C-HEGA-10, HEGA-10, C12E8, UDM, DM, NM and NG and PaPPase in DM, NM and OG ( and ). Of these DM gave the best improvement in diffraction resolution for both proteins, with some TmPPase crystals diffracting to 5 Å and some PaPPase crystals to 5.8 Å ( and ). To improve the resolution of the crystals further, we carried out the same optimization of crystallization conditions with DM purified proteins as we had done with DDM purified proteins, except that we did not test different PEGs or different salt concentrations. In addition to this, we tested crystallization by a modified version of the ‘HiLiDe'-lipid addition method (Gourdon et al. Citation2011). The only optimization step that proved to be beneficial was the addition of 0.1 mg/ml of DOPC, which gave a modest improvement in the resolution of TmPPase DM diffraction: the crystal diffracted to 4.3 Å () with a full data-set processable to 5 Å (unpublished work, T.K.).
Table III. Crystallization of TmPPase after detergent exchange.
Table IV. Crystallization of PaPPase after detergent exchange.
In a second round of detergent exchange, we tested detergent CyFos-6, FosC-12, D-thio-M, CHAPSO, CYM-6 and CYM-5 with TmPPase and CHAPSO, CYM-5 and CYM-6 with PaPPase. Detergent exchanges to CYM-6 and CYM-5 both increased the resolution of TmPPase diffraction to 3.9 Å ( and , ) with full data-sets processable to 4.2 Å (CYM-6, unpublished work, T.K) and 4 Å (CYM-5, Kellosalo et al. Citation2012). PaPPase did not crystallize in any of the second round detergents (). Optimization of crystallization conditions of TmPPase in CYM-6 or CYM-5 with similar methods as employed with TmPPase in DDM did not improve the resolution of crystal diffraction.
Figure 1. TmPPase CYM-6 and OGNPG crystals from sitting-drop vapour diffusion experiments. (A) The crystals were grown at 21°C in drops containing 0.2 μl of 10 mg/ml TmPPase in 20 mM MES-NaOH pH 6.5, 3.5% (v/v) glycerol, 50 mM KCl, 5 mM MgCl2, 1 mM Na2HPO4, 2 mM DTT and 0.14% CYM-6 and 0.2 μl of well solution containing 18% PEG 3350 MME and 0.64 M Na-Acetate pH 4.6. The reservoir contained 80 μl of well-solution. (B) The crystals were grown at 4°C in drops containg 1 μl of 10 mg/ml TmPPase in 20 mM MES-NaOH pH 6.5, 3.5% (v/v) glycerol, 50 mM KCl, 5 mM MgCl2, 1 mM Na2HPO4, 2 mM DTT and 1% OGNPG and 1 μl of well solution containing 19% PEG 350 MME, 100 mM MES-NaOH pH 6.5, 0.2 M CaCl2 and 2 mM DTT. The reservoir contained 1 ml of well-solution.
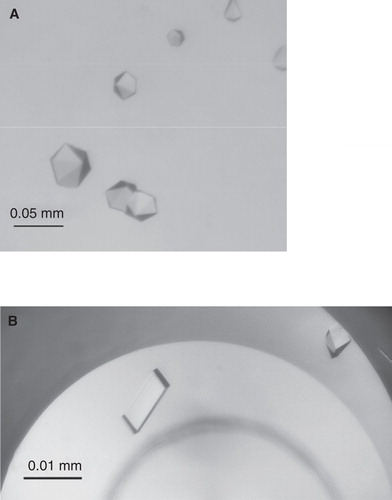
Most of the TmPPase crystals grown after the first and second round of detergent exchanges were in space group P21 (). The other crystal forms we found were P422-type primitive tetragonal for a TmPPase DM crystal and P6-type primitive hexagonal for TmPPase CYM-6 crystals, but the final assignment of the exact space group was not possible. Addition of 0.1 mg/ml of DOPC to the crystallization conditions of TmPPase in DM changed the space group from P422-type to P21 (). The crystals of TmPPase in CYM-6 grew in the presence of PEG 3350 while all the other crystals were grown with PEG 400 as the precipitant (). The use of this higher molecular weight PEG could be the reason for the difference in the space group of the TmPPase CYM-6 crystals. Crystals of PaPPase in DM were in space group C222 or C2221 ().
Crystals of TmPPase in DM, CYM-6 and CYM-5 suffered from similar problems as the DDM crystals: We could not reproduce the 3.9 Å diffracting TmPPase CYM-5 or CYM-6 crystals even with protein from the same batch and the crystallization behaviour of the protein varied from drop to drop. As with DDM, the optimal concentration of CYM-6, CYM-5 and DM for crystallization of either TmPPase or PaPPase was ≈5 × CMC (0.45% DM, 0.6% CYM-5, 0.14% CYM-6). TmPPase did crystallise from ≈2 × CMC CYM-6, CYM-5 or DM (0.175% DM, 0.06% CYM-6, 0.24% CYM-5), but none of these crystals diffracted as well as the best crystals grown with higher detergent concentration (). Altogether we harvested several hundreds of crystals from different conditions and took these to the ESRF and SLS for X-ray diffraction measurements, most of which diffracted poorly to less than 10 Å (see Methods).
Optimization of TmPPase by detergent exchange to OGNPG
In the third round of detergent exchanges we tested CYM-4, DMNPG and OGNPG with TmPPase and OGNPG with PaPPase. PaPPase did not crystallize in this detergent (), but the use of 1% OGNPG (≈17 × CMC) gave a marked improvement in the resolution of TmPPase diffraction (). The 3.2 Å resolution of initial TmPPase OGNPG crystals (measured at ID23-1 beam-line at ESRF) was improved even further with bigger crystals () grown in 2 μl drops and matching the beam size to the crystal size, and our current best data set (), collected at I911-3 beamline of MAX-lab extends to 2.6 Å () and is more isotropic in quality than any previous data set. The crystals grew reproducibly in space group P21, and a plot of the self rotation function of the collected data shows as expected as single non-crystallographic two-fold symmetry peak due for the TmPPase dimer at ϕ = −63.4°, ϕ = 0°, χ = 180°, while a second symmetry equivalent peak is generated 90° from this due to combination of non-crystallographic and crystallographic symmetry ().
Table V. Statistics of a data-set collected from TmPPase OGNPG crystal.
Figure 3. A χ = 180° plot of the self-rotation function from the 2.6 Å data set of TmPPase OGNPG crystal. The self-rotation search was done at 4.5Å resolution with a 30 Å search radius. The unique b-axis is plotted horizontal, and the non-crystallographic peak describing the dimer in the asymmetric unit, at 8.3 σ, occur at ϕ = −63.4°, ϕ = 0°, while the peak at ϕ = 26.4°, ϕ = 0°, is generated by the perpendicular 2-fold in the rotation function, and the lower peaks are generated apparently by the helical assembly.
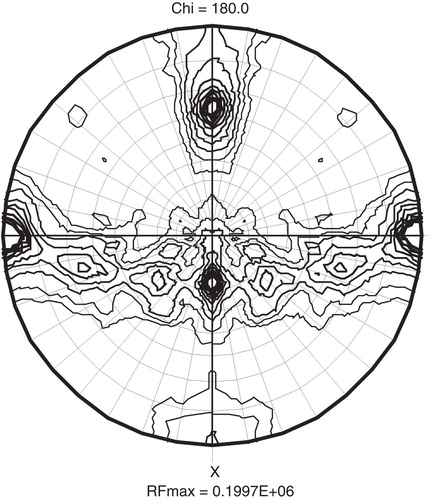
Discussion
In this study we present the crystallization of two recombinantly produced membrane-bound pyrophosphatases: A sodium pump from T. maritima and a proton pump from P. aerophilum. We also present our results on the crystallization of TmPPase that has allowed us to grow crystals diffracting to a resolution of 2.6 Å ( and ).
The decisive step in optimizing the crystal growth was the exchange of the detergent DDM used in the solubilization of the protein during the Ni-affinity purification step. A similar kind of detergent exchange scheme has been widely employed in the crystallization of membrane proteins (see e.g., Ostermeier et al. Citation1997, Lemieux et al. Citation2003, Screpanti et al. Citation2006).
Even after thorough optimization, we could only grow poorly diffracting crystals of TmPPase or PaPPase in DDM-based detergent mixtures, with the best diffraction from TmPPase crystals being highly anisotropic and extending to 4 Å in one direction, but only to 8 Å in the other (), while we could only obtain crystals of PaPPase diffracting to 9 Å. This is not surprising as the large micelle size of DDM seems to make it a poor detergent for crystallization (Sonoda et al. Citation2010, Citation2011), even though proteins are usually active in it. The resolution of the diffraction of TmPPase and PaPPase was improved to 5 Å and 6 Å, respectively, with the exchange of the detergent to DM. The resolution of the TmPPase DM crystals was improved further to 4.3 Å by the addition of lipid DOPC according to the ‘HiLiDE'-method (Gourdon et al. Citation2011). The resolution of TmPPase diffraction was improved to 3.9 Å with exchange to CYM-6 or CYM-5 ( and , ).
The decisive step, however, in the improvement of TmPPase crystal quality came through detergent exchange in to OGNPG, a neopentyl glycol detergent (Chae et al. Citation2010) that has only recently become available. This step not only resulted in improvement in diffraction quality with the best crystal diffracting to 2.6 Å (), but also improved the reproducibility of the crystallization. With DDM, DM, CYM-5 and CYM-6, TmPPase crystals even from identical drops had widely differing qualities, but with OGNPG we have been able to grow reproducibly crystals diffracting to at least 3 Å. It is also worth noting that microbeam scanning of the crystals and use of highly intense beam from third generation synchrotrons such as ERSF and Diamond did not improve to resolution compared to Max-Lab II 911-3 beam line for the OGNPG crystals (unpublished work, T.K). The best data were collected at Max-Lab II, by carefully matching the crystal and beam size.
With most of the detergents, the optimal crystallization conditions required detergent concentrations that were ≈4 × CMC or above. In the case of DM, CYM-5 and CYM-6 these concentrations are around 2-fold higher than the ones used for crystallization of several other membrane proteins (see e.g., Ostermeier et al. Citation1997, Jiang et al. Citation2002, Long et al. Citation2010). The reason for the beneficial effect of the higher detergent concentrations for the crystallization of TmPPase might be that it makes the detergent exchange more effective during the Ni-affinity step. Alternatively, it might be that at these concentrations beneficial detergent-detergent interactions take part in packing the M-PPases in the crystal lattice (Loll Citation2003). Packing of TmPPase OGNPG inside the crystal () is of the type I (Loll Citation2003), which supports the crucial role of the detergent-detergent interactions in facilitating the creation of layered 2D-crystalline sheets.
Figure 4. TmPPase packing in the high resolution OGNPG crystal form. The electron density of TmPPase molecules shows type I crystal packing. The structure determination is published by Kellosalo et al. (Citation2012).
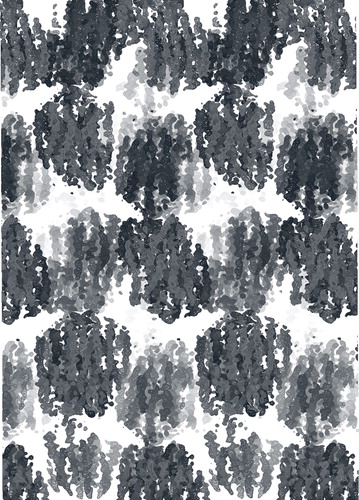
With TmPPase low pyrophosphatase activity of the protein in a detergent was strongly correlated with poor crystallization behaviour; TmPPase C12E8, CHAPSO, CyFos-6 or FosC-12 had only ca. 20% of the activity of TmPPase DDM () and did not produce crystals (). TmPPase C-HEGA had 10% of the activity of TmPPase DDM () and while it did produce crystals these did not diffract (). With other detergents in which TmPPase had over of 80% of the activity of TmPPase DDM there was no correlation between activity and crystallization behaviour: TmPPase CYM-5 and CYM-4 which had 85% and 80% of TmPPase DDM activity (), respectively, produced better diffracting crystals than TmPPase UDM () which had the same activity as TmPPase DDM (). This is probably due to the importance of other detergent characteristics, such as small micellar size and detergent-detergent interactions (Loll Citation2003), over the stabilizing effect of the detergent. PaPPase showed similar activity in all of the eight tested detergents (), but it crystallized in only two of these with the best crystals diffracting only to 5.8 Å (). The poor crystallization behaviour of PaPPase might be related to loss of activity during the purification as we could recover only 80% of the total activity after solubilisation (Kellosalo et al. Citation2011).
All TmPPas and PaPPase crystals grew from conditions that contained PEG as a precipitant (, and ), with well diffracting TmPPase OGNPG crystals growing with PEG 350 MME (). Small molecular PEGs like PEG 350 MME have been so far the most successful precipitants in crystallizing membrane proteins (Newstead et al. Citation2008).
TmPPase crystallized in at least four different crystal forms ( and ). For PaPPase, one crystal form was seen in which the crystals had either C222 or C2221 space group (). TmPPase DDM crystals had either P222 and/or P212121 or P422 space groups depending on the buffer (Novelle buffer 4 or Citrate), salts (0.1 M NaCl2 and 0.1 M Li2SO4 or 0.1 M NaCl) and the presence of additional detergents (0.56% NG or 1% CHAPSO) in the crystallization conditions (). When TmPPase was crystallized after detergent exchange we obtained crystals with space groups P422, P21 and P6 (). Similar behaviour in which the presence of a different detergent affects the crystal form in which the membrane protein crystals grow has also been seen before (Lemieux et al. Citation2003, Khademi et al. Citation2004, Zheng et al. Citation2004).
After this paper was in review, a structure of a proton-pumping M-PPase of Vigna radiata (VrPPase) was published (Lin et al. Citation2012). This protein was crystallized in the presence of a substrate analogue, imidodiphosphate (IDP), and its 2.35 Å resolution structure shows the IDP binding to the active site of the enzyme. The new well diffracting crystals of TmPPase described here have now allowed us to determine the structure of the TmPPase enzyme at high resolution (Kellosalo et al. Citation2012). These structures (Kellosalo et al. Citation2012) show the enzyme in either phosphate-bound or resting, metal bound state. TmPPase crystallized in metal bound state in crystallization conditions where the phosphate of the crystallization buffer (see Crystallization of TmPPase and PaPPase section) precipitated due to the high concentration of calcium (0.2 M) in the well solution (). In general, the structure of the VrPPase and TmPPase is the same, and the structures have allowed us to derive a catalytic model (Kellosalo et al. Citation2012).
M-PPases have no apparent sequence or structural similarity to other phosphatase ion-pumps, and so the TmPPase crystals obtained will help in unravelling a novel catalytic mechanism for phosphorolysis assisted ion-transport.
Conclusions
Membrane-bound pyrophosphatases (M-PPases) are membrane proteins that couple pyrophosphate hydrolysis to Na+ or proton pumping (Malinen et al. Citation2007). Due to the scarcity of structural information, the mechanism of catalysis of these enzymes is poorly understood. In this paper we present the results of our crystallization experiments, which have allowed us to grow crystals of two M-PPases: Of a sodium pump of T. maritima (TmPPase) and of a proton pump of P. aerophilum (PaPPase). Optimization of TmPPase crystals which has included exchange of dodecyl maltoside used in the solubilization of the enzyme to octyl glucose neopentylglycol and crystallization in 2 μl, rather than the original 0.4 μl drops, has allowed us to grow crystals diffracting to 2.6 Å. These crystals now allow the determination of the structure, which will shed light on the little understood mechanism of M-PPase catalysis.
Acknowledgements
We acknowledge the assistance received from the Biocenter Finland crystallization facility, the excellent technical help provided by D. Bansfield and S. Mäki, the generous gift of plasmid pRS1024 from Professor A. Serrano (University of Sevilla), the generous gift of S. cerevisae BJ1991 cells from Ineke Braakman (Utrecht University), the generous gift of T. marítima expression plasmid and scientific advice from Prof. R. López-Marqués (University of Copenhagen) and the crucial help from Drs K. Kogan and R. Kolodziejczyk. We also acknowledge the advice given by Sir J. Walker (Medical Research Council) on testing the neopentyl glycol detergents and the advice and help given by Prof. Poul Nissen (Aarhus University), Dr Pontus Gourdon (Aarhus University) and Dr Maria Nyblom (Aarhus University) on the ‘HiLiDe'-method. We acknowledge the European Synchrotron Radiation Facility, MaxLab in Lund and Swiss Light Source and the various beamline scientists, and in particular Dr Marjolein Thunnissen at Max-lab and Dr Matthew Bowler at the ESRF. Funding: The authors acknowledge financial support from the European Drug Initiative on Channels and Transporters (EDICT, Grant number 400707), the Academy of Finland (Grant numbers 114752 and 1115224) to AG and TK and the National Graduate School of Informational and Structural Biology (ISB) to JK.
Declaration of interest: The authors report no conflicts of interest. The authors alone are responsible for the content and writing of the paper.
References
- Chae PS, Rasmussen SGF, Rana RR, Gotfryd K, Chandra R, Goren MA, 2010. Maltose-neopentyl glycol (MNG) amphiphiles for solubilization, stabilization and crystallization of membrane proteins. Nat Methods 7:1003–1008.
- Garcia-Contreras R, Celis H, Romero I. 2004. Importance of Rhodospirillum rubrum H+-pyrophosphatase under low-energy conditions. J Bacteriol 186:6651–6655.
- Gourdon P, Andersen JL, Hein KL, Bublitz M, Pedersen BP, Liu XY, 2011. HiLiDe-systematic approach to membrane protein crystallization in lipid and detergent: Published as part of the Crystal Growth & Design virtual special issue on the 13th International Conference on the Crystallization of Biological Macromolecules (ICCBM13). Cryst Growth Des 11:2098–2106.
- Iwata S. 2003. Methods and results in crystallization of membrane proteins. La Jolla, CA: International University Line.
- Jiang Y, Lee A, Chen J, Cadene M, Chait BT, MacKinnon R. 2002. Crystal structure and mechanism of a calcium-gated potassium channel. Nature 417:515–522.
- Kabsch W. 1993. Automatic processing of rotation diffraction data from crystals of initially unknown symmetry and cell constants. J App Cryst 26:795–800.
- Kellosalo J, Kajander T, Kogan K, Pokharel K, Goldman A. 2012. The Structure and Catalytic Cycle of a Sodium-Pumping Pyrophosphatase. Science. [EPub ahead of print]
- Kellosalo J, Kajander T, Palmgren MG, Lopéz-Marqués RL, Goldman A. 2011. Heterologous expression and purification of membrane-bound pyrophosphatases. Protein Express Purif 79:25–34.
- Khademi S, O'Connell J, Remis J, Robles-Colmenares Y, Miercke LJW, Stroud RM. 2004. Mechanism of ammonia transport by Amt/MEP/Rh: Structure of AmtB at 1.35 Å. Science 305:1587–1594.
- Lemercier G, Dutoya S, Luo S, Ruiz FA, Rodrigues CO, Baltz T, 2002. A vacuolar-type H+-pyrophosphatase governs maintenance of functional acidocalcisomes and growth of the insect and mammalian forms of Trypanosoma brucei. J Biol Chem 277:37369–37376.
- Lemieux MJ, Song J, Kim MJ, Huang Y, Villa A, Auer M, 2003. Three-dimensional crystallization of the Escherichia coli glycerol-3-phosphate transporter: A member of the major facilitator superfamily. Prot Sci 12:2748–2756.
- Lin SM, Tsai JY, Hsiao CD, Huang YT, Chiu CL, Liu MH, 2012. Crystals structure of membrane-embedded H+-translocating pyrophosphatase. Nature 484:399–403.
- Loll PJ. 2003. Membrane protein structural biology: The high throughput challenge. J Struct Biol 142:144–153.
- Long F, Su CC, Zimmermann MT, Boyken SE, Rajashankar KR, Jernigan RL, 2010. Crystal structures of the CusA efflux pump suggest methionine-mediated metal transport. Nature 467:484–488.
- López-Marqués RL, Pérez-Castiñeira JR, Buch-Pedersen MJ, Marco S, Rigaud JL, Palmgren MG, 2005. Large-scale purification of the proton pumping pyrophosphatase from Thermotoga maritima: a ‘Hot-Solve' method for isolation of recombinant thermophilic membrane proteins. Biochim Biophys Acta 1716:69–76.
- Lopéz-Marqués RL, Péréz-Castineira JR, Losada M, Serrano A. 2004. Differential regulation of soluble and membrane-bound inorganic pyrophosphatases in the photosynthetic bacterium Rhodospirillum rubrum provides insights into pyrophosphate-based stress bioenergetics. J Bacteriol 186:5418–5426.
- Maeshima M. 2000. Vacuolar H+-pyrophosphatase. Biochim Biophys Acta 1465:37–51.
- Malinen AM, Belogurov GA, Baykov AA, Lahti R. 2007. Na+-pyrophosphatase: A novel primary sodium pump. Biochemistry 46:8872–8878.
- McIntosh MT, Vaidya AB. 2002. Vacuolar type H+ pumping pyrophosphatases of parasitic protozoa. Int J Parasitol 32:1–14.
- McIntosh MT, Drozdowicz YM, Laroiya K, Rea PA, Vaidya AB. 2001. Two classes of plant-like vacuolar-type H+-pyrophosphatases in malaria parasites. Mol Biochem Parasitol 114:183–195.
- Mimura H, Nakanishi Y, Hirono M, Maeshima M. 2004. Membrane topology of the H+-pyrophosphatase of Streptomyces coelicolor determined by cysteine-scanning mutagenesis. J Biol Chem 279:35106–35112.
- Newman J. 2004. Novel buffer systems for macromolecular crystallization. Acta Crystallogr D D60:610–612.
- Newstead S, Ferrandon S, Iwata S. 2008. Rationalizing alpha-helical membrane protein crystallization. Prot Sci 17:466–472.
- Ostermeier C, Harrenga A, Ermler U, Michel H. 1997. Structure at 2.7 Å resolution of the Paracoccus denitrificans two-subunit cytochrome c oxidase complexed with an antibody FV fragment. Proc Natl Acad Sci USA 94:10547.
- Screpanti E, Padan E, Rimon A, Michel H, Hunte C. 2006. Crucial steps in the structure determination of the Na+/H+ antiporter NhaA in its native conformation. J Mol Biol 362:192–202.
- Serrano A, Pérez-Castineira JR, Baltscheffsky M, Baltscheffsky H. 2007. H+-PPases: Yesterday, today and tomorrow. IUBMB Life 59:76–83.
- Sonoda Y, Cameron A, Newstead S, Omote H, Moriyama Y, Kasahara M, 2010. Tricks of the trade used to accelerate high-resolution structure determination of membrane proteins. FEBS Lett 584:2539–2547.
- Sonoda Y, Newstead S, Hu NJ, Alguel Y, Nji E, Beis K, 2011. Benchmarking membrane protein detergent stability for improving throughput of high-resolution X-ray structures. Structure 19:17–25.
- Zheng L, Kostrewa D, Berneche S, Winkler FK, Li X-D. 2004. The mechanism of ammonia transport based on the crystal structure of AmtB of Escherichia coli. Proc Natl Acad Sci USA 101:17090–17095.