Abstract
Objective: In this study, pyrazinamide (PZA)-proliposome in a dry powder aerosol form was developed for delivering drugs to alveolar macrophages (AMs) infected with mycobacteria.
Materials and methods: PZA-proliposomes consisting of pyrazinamide, soybean phosphatidylcholine, cholesterol and porous mannitol were prepared by a spray drying method. The PZA-proliposome physicochemical properties were determined using a cascade impactor, X-ray diffraction, differential scanning calorimetry and infrared spectroscopy. The toxicity of proliposomes to respiratory-associated cell lines (Calu-3, A549 and NR8383) and its potential to provoke immunological responses from AMs were determined. In vivo repeated dose toxicity in rats was evaluated.
Results and discussion: PZA-proliposomes were successfully prepared. For the aerosolization properties of PZA-proliposomes at 60 l/min, the powders showed mass median aerodynamic diameters of 4.26–4.39 µm, with fine particle fractions (aerosolized particles less than 4.4 µm) of 20–30%. Encapsulation of PZA was 26–45%. PZA-proliposomes were less toxic to respiratory-associated cells, and did not activate AMs to produce inflammatory mediators, including interleukin-1β, tumor necrosis factor-α, and nitric oxide, at a toxic level. Renal and liver toxicity in rats were not observed.
Conclusions: We suggest that PZA-proliposomes are potential candidates for pulmonary tuberculosis treatment.
Introduction
The use of alveolar delivery systems are an attractive approach for delivering drugs to lungs of tuberculosis (TB) patients especially to access and inhibit infected alveolar macrophages (AMs) (Alving, Citation1998; Ahsan et al., Citation2002). These systems have many benefits such as being target oriented, low dose, with low side effects and less frequent administration (Mitchison and Fourie, Citation2010). The important outcomes are better drug compliance, reduced drug resistance and shorter courses of therapy. Pulmonary administration is an appropriate route for drugs required to have a local action in the respiratory tract as it results in rapid localized drug action. One major advantage of using liposomes for pulmonary administration is that they can be prepared from phospholipids that are normal components of lung tissues. It can therefore be assumed that pulmonary-delivered liposomal vesicles will lack inherent toxicity (Taylor and Elhissi, Citation2006). The technology for lung specific drug delivery systems is now at a point where aerosolized liposomes and even timed release methodology may offer advantages for a more effective treatment and prevention of TB (Khuller et al., Citation2004). Nebulization of liposomes would be perhaps more acceptable when compared with I.V. liposomes. Pandey and co-workers (Citation2004) have suggested that the controlled drug release together with macrophage targeting may obviate the need for daily anti-TB drug dosing and consequently, liposome based inhaled therapy would be a novel approach towards the management of pulmonary TB. Liposomes are relatively unstable colloidal systems. Physical instability is manifested in vesicle aggregation and fusion, associated with changes in vesicle size and loss of entrapped hydrophilic materials (Elhissi et al., Citation2007). Chemical instability, particularly ester bond hydrolysis, and oxidation of unsaturated acyl bonds accelerates liposome breakdown and alters the drug-release characteristics (Heurtault et al., Citation2003). Since, the therapeutic efficacy of liposomes is dependent upon the physical integrity and stability of lipid bilayer structures; it may become imperative that the chemical and physical stability of liposomes is maintained (Khuller et al., Citation2004). To overcome instability problems, proliposome approaches to liposome formation have been described as a means for enhancing stability. Freeze dried and spray dried liposomes can be a useful tool to prepare proliposomes (Taylor and Elhissi, Citation2006; Agnihotri et al., Citation2010). Proliposomes are defined as dry, free-flowing particles that immediately form a liposomal dispersion on contact with water, so they have a potential for pulmonary delivery as dry-powder inhalers (DPIs) (Elhissi et al., Citation2010; Wagner and Vorauer-Uhl, Citation2011). Anti-TB proliposomes have been studied (Changsan et al., Citation2009a). Rifampicin proliposomes were prepared by freeze-drying with mannitol as a cryoprotectant (Changsan et al., Citation2009b). These encapsulated rifampicin liposomes were evidently nontoxic to respiratory associated cells, including bronchial epithelial cells, small airway epithelial cells and AMs. The minimum inhibitory concentration against Mycobacterium bovis was 0.2 μM for proliposomes containing rifampicin and 0.8 μM for free rifampicin. In addition to freeze-drying, using a spray drying method is an alternative approach (Rojanarat et al., Citation2011). Isoniazid (INH) proliposomes in a dry powder aerosol form were prepared by a spray drying method. These INH proliposomes were nontoxic to respiratory-associated cells, and did not activate AMs to produce inflammatory mediators, including interleukin-1β (IL-1β), tumor necrosis factor-α (TNF-α), and nitric oxide, at a toxic level. The efficacy of INH proliposome against AMs infected with M. bovis was significantly higher than that of free INH. The authors indicated that anti-TB drugs entrapped in proliposome enhanced drug bioactivity and were potential candidates for an alternative TB treatment (Rojanarat et al., Citation2011).
In this study, pyrazinamide (PZA)-proliposomes were prepared by a spray drying method using porous mannitol which had a low density and high porosity. The toxicity of proliposome formulations with good aerodynamic properties against respiratory and macrophage cell lines was investigated. Phagocytosis by AMs incubated with proliposomes was observed. The in vivo repeated dose toxicity of proliposome was investigated in Wistar rats administered with proliposomes by intratracheal instillation.
Materials and methods
Materials
Mannitol, L-α soybean phosphatidylcholine (SPC), and cholesterol from lanolin (CH) were obtained from Fluka (Buchs, Switzerland). Ammonium carbonate was from Ajax Finechem (NSW, Australia). Pyrazinamide (PZA) was from Sigma-Aldrich (St. Louis, MO, USA), and dimethyl sulfoxide (DMSO) from Riedel-de Haën (Seelze, Germany). All other reagents were analytical grade.
All solutions (reagent packs) used for the maintenance and culture of human bronchial epithelial cells (Calu-3) and human lung adenocarcinoma cell line (A549) were acquired from Gibco (Grand Island, NY, USA). Samples of 3-(4, 5-dimethylthiazol-2-yl)-2,5-diphenyltetrazolium bromide (MTT) and fluorescein diacetate (FDA) were from Sigma-Aldrich. Quantikine® RTA00 and Quantikine® RLB00 kits for rat TNF-α and IL-1β, respectively, were from R&D Systems Inc. (Minneapolis, MN, USA). The BCG vaccine of Mycobacterium bovis (M. bovis), Middlebrook 7H9 culture medium, Mycobacterium tuberculosis H37Ra (ATCC 25177) cells and alamar blue solution were supplied by the Queen Saovabha Memorial Institute (Bangkok, Thailand), Becton Dickinson and Co. (Franklin Lakes, NJ, USA), American Type Culture Collection (Rockville, MD, USA) and Alamar Biosciences/Accumed (Westlake, OH, USA), respectively. Zoletil®100 was from Virbac Laboratories (Carros, France).
Production of porous mannitol
Mannitol and ammonium carbonate in a weight ratio of 4:1 were dissolved in distilled water. The total solid concentration in solution was 10 mg/ml. The solution was sprayed through a 1.5 mm nozzle using a mini spray dryer B-290 (Büchi, Flawil, Switzerland) at an inlet temperature of 110°C, an atomizing pressure of 800 kPa, and a feeding rate of 3 ml/min. The product was separated and collected by the cyclone and then directed into the collecting chamber. A porous mannitol preparation was produced.
Production of PZA-proliposome by spray-drying technique
To prepare the PZA-proliposome formulations, the ingredients were divided into two parts. The lipid part was composed of a mixture of SPC (0.06 mmole) and CH (0.06 mmole) (Changsan et al., Citation2009a). The porous mannitol used as a core carrier and PZA was the powder part. The powder part was calculated to be 10 mg/ml and the weight ratio of PZA: porous mannitol was 1:9 (Formulation I), 2:8 (Formulation II), 4:6 (Formulation III), 6:4 (Formulation IV) and 8:2 (Formulation V).
The lipid part was weighed and dissolved in 100 ml of 95% ethanol to obtain the ethanolic lipid solution. PZA was added to the ethanolic lipid solution and sonicated until a clear solution was obtained. The maximum concentration of PZA in this study was only 0.8% and was completely dissolved. Porous mannitol was dispersed in the solution, and the suspension was sonicated for 15 min in order to deaggregate mannitol particles before the spray drying process began. The suspension was continuously stirred to provide homogeneity of the suspension during spray drying. The inlet temperature was 90°C and the atomizer pressure was 800 kPa, with a feed rate of 3 ml/min. The proliposome powder was collected from the collecting chamber and kept in a desiccator until used.
Morphology of the microparticles and PZA-proliposomes
The samples were sprinkled on an aluminum stub and then coated with gold by a sputtering technique using a Sputter Coater (SPI supply, USA) in an argon atmosphere for 60 s. The surface morphology of the microparticles was examined by scanning electron microscopy (SEM) (FEI Quanta 400 FEG, FEI Company, USA) at 20 kV.
Content uniformity of PZA in the proliposome powder
The PZA-proliposome powder (10 mg) was randomly sampled and weighed. The powder samples were suspended in 10 ml of methanol to dissolve the lipid content. The volume was adjusted to 25 ml with distilled water, followed by sonication for 15 min to obtain a clear solution. The PZA content from the clear solutions was analyzed by high performance liquid chromatography (HPLC). The HPLC system was equipped with an AS 3000 autosampler, a P1000 pump and a UV 2000 detector (Thermo Fisher Scientific, Waltham, MA, USA). A microbondapak C18 column (Phenomenex, Torrance, CA, USA) (250 × 4 mm i.d., 5 µm) was used as a stationary phase. The mobile phase was phosphate buffer:acetonitrile (97:3 v/v), running at a flow rate of 1 ml/min. UV detection was at 254 nm.
In vitro evaluation of aerosol performance of the PZA-proliposome dry powder using a cascade impactor
The PZA-proliposome dry powder was aerosolized using a delivery device made in-house (Srichana et al., Citation2003). The aerosolized parameters of the products, including mass median aerodynamic diameter (MMAD), fine particle fraction (FPF) and emitted dose (ED), were determined using an Andersen cascade impactor (ACI) (Andersen Instruments, Atlanta, GA, USA) equipped with preseparator and glass throat. The ACI used a vacuum pump with a flow rate of 60 l/min for 10 s. Triton X-100 (0.1%) in methanol was used to rinse particles deposited on each stage. The PZA content was determined by HPLC, as described in the content uniformity section. The cumulative percentage of deposition was transformed to a Z-value and plotted against the log cutoff diameter of each stage. MMAD was obtained from the particle diameter at a Z-value of zero (Atkins et al., Citation1992). The emitted dose is the amount of drug propelled from the delivery device. The FPF is the fraction of particles smaller than 4.4 µm.
Encapsulation and size measurement after reconstitution of PZA-proliposome into a liposome suspension
To determine the percent encapsulation, 10 mg of proliposome powder was reconstituted with distilled water (4 ml), and then centrifuged with a SW 60 Ti rotor (Beckman Coulter Inc., Palo Alto, CA, USA) at 100,000 g for 20 min at 25°C (Saetern et al., Citation2004). The supernatant was analyzed as the unencapsulated PZA by HPLC, as described in the content uniformity section. The percent encapsulation was obtained using the following equation:
The size of the liposome after reconstitution was measured using ZetaPALS (Brookhaven, New York, USA) at 25°C. The proliposome powder was reconstituted with milliQ water to obtain nanovesicles while the undissolved particles were removed by centrifugation. The centrifugation conditions were 10,000 g for 20 min at 25°C. Sizes were determined immediately after obtaining the supernatant from the centrifugation.
X-ray diffraction measurement of PZA-proliposome
An X-ray diffraction (XRD) of the porous mannitol, PZA and PZA-proliposome was performed with a Siemens D 5000 (Siemens AG, Berlin, Germany) equipped with a diffracted-beam monochromator, using Cu radiation. The proliposome powder samples were spread over glass sample holders, each in an area of 4 cm2 with a depth of 1 mm. The powder surfaces were pressed and smoothed with a glass slide. The diffraction intensity was recorded at an angle of 2θ from 5 to 60° with a step size of 0.05° and step time of 1 s. The total time of the diffraction scan was 19 min, and each sample was examined in three separate experiments. The voltage and current generator were set at 40 kV and 30 mA, respectively. The obtained data were analyzed by EVA software. Relative crystallinity was determined from the XRD results by the ratio of the intensity of a characteristic crystalline peak to that of the amorphous halo for each powder sample. If the ratio of the peak to the amorphous halo around it decreased, then the crystallinity was proportionately lower (Morris and Rodriguez-Hornedo, Citation1993).
Differential scanning calorimetry of PZA-proliposome
A differential scanning calorimetry (DSC) Saetern model 2920 (TA Instruments, Newcastle, DE, USA) was used to investigate the interaction of the PZA-mannitol-SPC in the proliposome powder produced by spray drying. A powder sample was placed in an aluminum pan, hermetically sealed, and then assessed by DSC from 30°C to 200°C at a rate of 10°C/min. The DSC thermograms were analyzed using the Universal Analysis 2000 program, version 3.4c.
Fourier transform-infrared spectroscopy (FT-IR) of PZA-proliposome
Infrared spectroscopy was used to characterize the functional groups of the samples. A dried sample was carefully mixed with dry KBr and pressed into a pellet. The solid pellet was placed in a magnetic holder. FT-IR spectra between 4000 and 400 cm−1 were determined after an accumulation of 16 scans using a Spectrum One (Perkin-Elmer, MA, USA).
Cell cultures
Growth of human bronchial epithelial cells (Calu-3), human lung adenocarcinoma cell line (A549) and alveolar macrophage cell line NR8383
The Calu-3 cell line (ATCC HTB-55, Rockville, MD, USA) was cultured in modified Eagle’s media supplemented with 10% fetal bovine serum (FBS), and 50 units/ml penicillin and 50 µg/ml streptomycin. The A549 cell line (ATCC CCL-185, Rockville, MD, USA) was cultured in Ham’s F12K supplemented with 10% FBS, 50 units/ml penicillin and 50 µg/ml streptomycin. Both cell lines were maintained at 37°C in a fully humidified atmosphere at 5% CO2 in air and the media were changed every 2–3 days. When the cells reached 60–80% confluency, they were rinsed with phosphate buffered saline solution (PBS) to wash off any remaining complex proteins that might neutralize the trypsin activity. PBS was aspirated, and the cells were covered with 2 ml of trypsin/EDTA solution. The cells were then detached from the plate by the trypsin/EDTA. Cells were centrifuged, resuspended and then transferred to a new culture flask. The medium was replaced with fresh medium two or three times weekly.
The NR8383 cells (ATCC CRL-2192, Rockville, MD, USA) had been isolated from normal rat lung lavage. The cells were cultured in F-12 Kaighn’s cell culture medium supplemented with 15% (v/v) heat-inactivated FBS, 50 units/ml penicillin, 50 µg/ml of streptomycin, and then incubated at 37°C, in 5% CO2 and 85% humidity. The cells were maintained by transferring floating cells to new flasks. Adherent cells could be harvested by scraping. Upon reseeding, about half of the cells re-attached. The medium was replaced with fresh medium two or three times weekly.
Determination of cytotoxicity of PZA-proliposome to cells in the respiratory tract
The viabilities of Calu-3, A549 and NR8383 cells were evaluated using the MTT assay to detect functioning mitochondria. Live mitochondria transform MTT to insoluble purple formazan crystals, which was measured with a spectrophotometer. Briefly, 100 µl of 1 × 105 cells/ml was cultured in each well of a 96-well plate and allowed to adhere and grow overnight at 37°C, in an environment containing 5% CO2 and 80% humidity incubator. The following day, the media (100 µl) was replaced with fresh media and 100 µl of cell culture media containing either PZA or PZA-proliposome was added. The plate was then incubated for 24 h. A filtered sterilized stock MTT solution (50 µl of 5 mg/ml in Dulbecco’s phosphate buffer saline, DPBS, Gibco, Grand Island, NY, USA) was added into each well containing 150 µl of fresh media and incubated for 4 h at 37°C. After that, the supernatant was carefully removed and the resulting formazan crystals were dissolved by adding 200 µl of dimethylsulfoxide (DMSO, Riedel-de Haën, Seelze, Germany) and thoroughly mixed. The absorbance was recorded at 570 nm with the microplate reader (Biohit BP 800, Helsinki, Finland). The proportion of viable cells in the treated well was compared to the untreated well.
Determination of the alveolar macrophage response to PZA-proliposome
Production of inflammatory cytokines
An enzyme linked immunosorbent assay (ELISA) method was used to measure the levels of the inflammatory cytokines TNF-α and IL-1β generated from NR8383 cells responding to PZA, PZA-proliposome or lipopolysaccharide (LPS) from E. coli (positive control). Commercial ELISA kits (Quantikine® RTA00 and Quantikine® RLB00 for rat TNF-α and IL-1β, respectively, R&D systems Inc., MN, USA) were used as described in the product assay procedures. The detectable amount of both TNF-α and IL-β was 5 pg/ml. Standards, controls and samples were pipetted into the wells and any rat TNF-α or IL-β present was bound by the immobilized antibody. After washing away any unbound substances, an enzyme-linked polyclonal antibody specific for rat TNF-α or IL-β was added into the wells. Following a wash to remove any unbound antibody-enzyme reagent, a substrate solution was added to the wells. The enzyme reaction yielded a blue product that turns yellow when the stop solution was added. The intensity of the color measured was in proportion to the amount of rat TNF-α or IL-β bound in the initial step. The sample values were then read from the standard curve.
Nitric oxide assay by the Griess reaction
NO released by NR8383 cells after being challenged with PZA, PZA-proliposome or LPS in a concentration range of 25–500 µg/ml for PZA and PZA-priliposome and 25–500 ng/ml for LPS was detected by the Griess reaction. NO in the form of nitrite (NO2−), which is one of the two primary, stable and nonvolatile products of NO was investigated. This measurement relies on a diazotization reaction of the Griess reagent. Griess reagent was prepared by mixing 1% sulfanilamide, 0.1% N-(1-naphthyl)-ethylenediamine dihydrochloride and 2.5% phosphoric acid in water. Equal volumes of cell supernatant (100 µl) and Griess reagent (100 µl) were mixed. After mixing for 10 min, the absorbance was determined using a microplate reader at 450 nm. The NO concentration was calculated from a sodium nitrite standard curve (Huttunen et al., Citation2000; Punturee et al., Citation2004).
Phagocytosis of anti-TB dry powder particles by macrophage cells
Proliposome dry powder (Formulation I) was reconstituted with distilled water to obtain 10 mg/ml. Quantum dot nanoparticles were employed for particle imaging (Lumidot®, Sigma-aldrich, St. Louis, USA). Lumidot® 640 (20 µg/ml) was added to the liposome suspension in a volume ratio 1:2. The mixture was sonicated for 3 min.
100 µl of 1 × 104 cells/ml NR8383 cells was cultured in each well of a 96-well plate and allowed to grow and adhere overnight at 37°C, in a 5% CO2 and 95% humidity incubator. Liposome (reconstituted Formulation I) stained with Lumidot® 640 was added. Phagocytosis of anti-TB particles by NR8383 cells was observed with a fluorescence microscope (Olympus, BX61, Olympus, Tokyo, Japan).
In vivo repeated dose toxicity study of the proliposomes
Male Wistar rats (230–290 g) used in this study were obtained from the Southern Laboratory Animal Facility, Prince of Songkla University, Hat Yai, Songkhla, Thailand. They were allowed to acclimatize to the conditions of the animal house for 1 week before the experiments and kept in a room maintained under standard environmentally controlled conditions of 22 ± 2°C and 12 h light–12 h dark cycle. They were supplied with standard rodent diets and water ad libitum. All procedures were reviewed and approved by the Animal Ethics Committees, Prince of Songkla University, Thailand (No. Ref 11/51). Wistar rats were randomly divided into a control and treatment group (n = 7 for each group). Before the samples were administered, a blood sample (100 µl) was collected for the pre-treatment samples using the tail vein sampling technique. The proliposome formulation I (4 mg/kg body weight) were administered by intratracheal instillation at day 1st followed by intratracheal inhalation from the 2nd day to the 28th day every day. Intratracheal instillation and inhalation were modified from previous study (Brain et al., Citation1976). Briefly, intratracheal instillation was performed after anesthetized by Zoletil®100 at 40 mg/kg body weight. Polyethylene tube No. 240 (Intramedic®, New Jersey, USA) 8 cm in length was inserted through the mouth to insert into tracheal and about 5 cm was left outside the oral cavity for drug administration. Proliposomes were loaded into the tube. When the rat inhaled, the proliposome powder traveled automatically into the rat airways. For intratracheal inhalation, the rats were allowed to breath through plastic tube (diameter = 1 cm, length = 8 cm) for at least 2 min, while proliposomes were blown from the other end. The blood samples (100 µl) were collected after being first administered for 7, 14, 21 and 28 days. Clinical signs for example food intake, feces, hair and behavior were observed once daily and body weights were recorded every week during the experiments. The collected blood was centrifuged at 2000 rpm for 10 min to obtain serum in separate tubes. Alanine aminotransferase (ALT), aspartate aminotransferase (AST), blood urea nitrogen (BUN) and creatinine (Cr) were evaluated for determination of liver and renal toxicity. All Serum biochemical parameters were measured with a Cobas Mira Plus Chemistry Analyzer.
Statistical analysis
Data, when applicable, are presented as a mean ± standard deviation (SD) from at least three samples unless indicated. The data were compared using analysis of variance (ANOVA) followed by a One-Way ANOVA to determine the difference between data sets. All statistical comparisons were calculated using SPSS software version 17 (SPSS Inc., Chicago, IL, USA). A p-value < 0.05 was considered statistically significant.
Results and discussion
Morphology of the mannitol microparticles and PZA-proliposomes
Porous particles had a similar geometric size in comparison to non-porous particles () but differed with respect to density. Therefore it is expected that the aerodynamic properties of the porous particles must be better than those of the non-porous particles. Spherical particles of porous mannitol with a size of around 1–3 µm () were obtained from the spray-dried mannitol and ammonium carbonate as a pore forming agent. Porous mannitol resulted from adding the pore forming agents that were later volatilized. This was similar to the method established by Nolan and co-workers (Citation2009). It was found that adding ammonium carbonate was necessary to obtain porous particles in water. The density of the porous particles (0.22 ± 0.02 g/ml) was significantly lower than that of a non-porous spray dried powder (0.53 ± 0.02 g/ml). Porous particles had nanopores on their surface with a porosity of 88.0 ± 4.0% from liquid displacement method.
Figure 1. The SEM images of (A) spray dried mannitol (bar = 1 µm), (B) porous mannitol (bar = 1 µm), (C–G) PZA- proliposome formulation I to V (bar = 3 µm) and (H) PZA (bar = 300 µm).
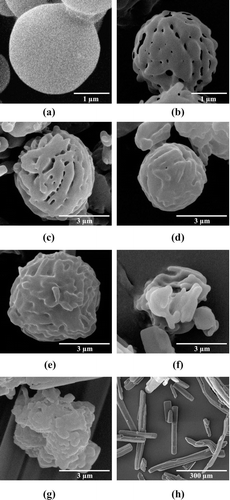
PZA appeared to be incorporated into the pores and on the surface of the core particles. PZA-proliposome powders were successfully prepared using the spray drying technique. The surface and morphology of the proliposomes obtained from formulations I to V are shown in –. Spherical microparticles were observed in formulation I, II and III (–) with a high (60–90%) content of porous mannitol. Other PZA-proliposomes containing porous mannitol less than 60% were irregular in shape, with some tiny particles (less than 1 µm) or elongated particles ( and ) adhering to large aggregated particles. In the spray drying process, the porous mannitol was dispersed in 95% alcohol containing PZA and the lipid part. PZA could be filled or adsorbed onto the porous mannitol and the particles became coated with the lipid. Formulation I, II and III had sufficient porous mannitol, so they were spherical in shape, while the formulation of IV and V were irregular. This might be due to insufficient amounts of porous mannitol in the formulation to produce spherical PZA-proliposome particles. The remaining drugs might be irregular in shape after the spray drying process. It could be expected that the liposomal properties of the formulation IV and V might be unsuitable from low emitted dose (<90%) and low percent encapsulation (<40%). Porous mannitol is an important ingredient to obtain spherical particles by the spray drying method. PZA-proliposome formulations were different in shape from the pure PZA (). This might be affected by the spray drying process that changed crystallinity and morphology. We have suggested that the ideal formulation should contain porous manitol of not less than 60%, to obtain perfect spheres. And other factors have to be taken into account such as MMAD, % encapsulation and FPF.
In vitro evaluation of the aerosol performance of the PZA-proliposome dry powder using the cascade impactor
For accuracy, both intra- and inter-day run gave the values of 99–100% recovery which were lined in the acceptable range. In the same way, the system precision was considered to be satisfactory since the % RSD values were less than 2% for both intra- and inter-day run. Linearity of analytical method was obtained between 0.5–10 µg/ml (R2 = 0.999, limit of detection = 13 ng/ml, limit of quantitation = 41 ng/ml) with a retention time of approximately 5 min. The presented methods are rapid, sensitive, and reproducible with good recovery values and therefore can be used for simultaneous quantification of PZA in different pharmaceutical formulations.
From , the uniformity of the drug content was acceptable (%RSD < 6) (USP 30 and NF 25; USP-NF, Citation2007). It indicated that the spray drying process produced a uniform distribution of the active ingredients throughout the PZA-proliposome products. All formulations showed their contents were in range of 93–109% of their labeled amount.
Table 1. Aerosolization properties, encapsulation and dynamic light scattering size measurement after reconstitution of PZA-proliposome formulations (mean ± SD, n = 3).
The PZA-proliposome formulations showed no difference in their MMAD values (4.26–4.39 µm, p > 0.05). The MMAD values were correlated with FPF, the fraction of particles smaller than 4.4 µm, in the range of 20–30%. An emitted dose (ED) of 80–98% was obtained from the formulations. FPF decreased when the porous mannitol increased until the porous mannitol reached 40%. In formulation IV and V containing low porous mannitol (40 and 20%, respectively), FPF was slightly increased. This might be from the lower size of the particles obtained from the spray drying. As a result, the uncoated PZA may travel deeper into lower stage of impactor. ED values decreased to lower than 90% when the porous mannitol ratio decreased to 40% or lower. This might be due to a greater aggregation of particles that caused difficulty in de-aggregating. Formulation I gave a higher FPF, with the highest ED value of 98%. To deliver the dry powder inhaler to the lower part of the respiratory tract, the MMAD of the formulation should be less than 5 µm (Thompson, Citation1992) and all prepared formulations met this requirement.
In this study, the proliposome density was modified using porous mannitol as core carrier. As a porous mannitol has a low density, it would improve the aerodynamic properties of a formulation. Particle’s aerodynamic diameter was directly proportional to square root of particle density and geometry of particle (Crowder et al., Citation2002). As particle density decreases, particle’s aerodynamic diameter decreases.
Encapsulation and size measurement after reconstitution of PZA-proliposome into a liposome suspension
After PZA-proliposomes were reconstituted with distilled water, vesicle sizes () showed a normal distribution. The mean size ranges were 200–520 nm from all formulations. Formulation I gave the lowest vesicle size (200 nm). Nanoparticles (200–600 nm) were efficiently taken up by macrophages (Kanchan and Panda, Citation2007). It is expected that the PZA-liposome in this study is suitable for targeting AM.
The encapsulation efficiency of PZA was in the range of 26–45% (). In this study, the encapsulation efficiency was determined using an indirect method. PZA-proliposome was reconstituted with distilled water, whereas free PZA would dissolve instantly. After ultracentrifugation, PZA-encapsulated liposomes were packed into pellets at the bottom of the centrifuge tube. The supernatant was analyzed to determine free PZA. The encapsulation efficiency was calculated from the difference between the PZA content and the free PZA values. Formulation I had the highest PZA encapsulation, while formulation V had the lowest. This could be explained by a low PZA loading amount in the formulation providing for high encapsulation efficiency. In the spray-drying process, PZA could be incorporated into the porous mannitol particles before the lipid coating. When the PZA loading was high (formulation IV and V), therefore the lipid could not coat all the particles. That is why the drug loading was kept at low level. Also, when the drug: lipid ratio was increased the percent encapsulation dramatically decreased. A PZA:porous mannitol ratio of 1:9 seemed to give the highest encapsulation efficiency.
X-ray diffraction measurement of PZA-proliposome
The XRD pattern of the porous mannitol, PZA-proliposome formulation I to V and PZA are shown in . The porous mannitol diffractogram showed sharp reflections at 2θ values of 14.58°, 16.76°, 18.70°, 20.38°, 21.06°, 21.67°, 23.35° and 29.43° (). In a similar way, the diffractogram of PZA showed reflections at 2θ values of 7.80°, 13.74°, 15.32°, 15.66°, 17.67°, 27.11°, 27.37° and 35.80° (). It was identified as polymorph α (Castro et al., Citation2010). The sharp reflections of the diffractogram of the porous mannitol and PZA confirmed the crystallinity of the ingredients.
Figure 2. The X-ray diffraction patterns of (A) porous mannitol, (B–F) PZA-proliposome formulation I to V (containing PZA 10, 20, 40, 60 and 80% w/w, respectively) and (G) PZA.
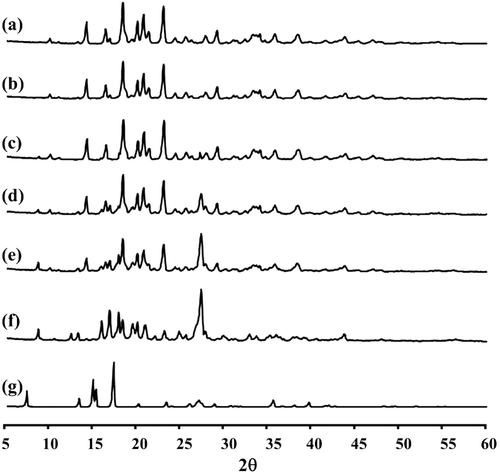
PZA-proliposome formulation I showed intense peaks of crystallinity at 14.58°, 16.76°, 18.71°, 20.39°, 21.07°, 21.67°, 23.35° and 29.44° (). This was the same XRD pattern for porous mannitol. Formulation II () exhibited intense peaks of crystallinity like the XRD pattern of porous mannitol (14.57°, 16.75°, 18.71°, 20.38°, 21.07°, 23.35°, 29.44° and 33.52°). Formulation III showed reflections at 2θ values of 14.57°, 16.75°, 18.71°, 20.39°, 21.06°, 23.36°, 27.64° and 29.43° (). The XRD pattern of formulation IV showed intense peaks of crystallinity at 14.58°, 17.25°, 18.22°, 18.70°, 20.38°, 21.07°, 23.38° and 27.65° (). The diffractogram of formulation V showed reflections at 2θ values of 16.31°, 17.23°, 18.24°, 18.69°, 19.79°, 20.37°, 21.31° and 27.65° (). Several new reflections appeared in the spectra (calculated dspacing in Angstroms) for example 33.52° (2.67 °A) for formulation II; 27.64° (3.22 °A) for formulation III; 17.25° (5.14 °A), 18.22° (4.87 °A) and 27.65° (3.23 °A) for formulation IV and 16.31° (5.44 °A), 17.23° (5.15 °A), 18.24° (4.86 °A), 19.79° (4.49 °A), 21.31° (4.17 °A) and 27.65° (3.23 °A) for formulation V.
The X-ray diffraction patterns of PZA-proliposome formulations II, III, IV and V were not matched to either mannitol or PZA. PZA-proliposome formulations II – V was unlikely to be polymorph α, β, δ or γ (Castro et al., Citation2010).
Differential scanning calorimetry of PZA-proliposome
DSC experiments were carried out to evaluate the thermal behavior of PZA-proliposomes in relation to the individual ingredients. The DSC thermograms and thermal data for porous mannitol, PZA-proliposome formulation I to V and PZA are presented in and . Porous mannitol exhibited an endothermic peak at 163.5°C and a melting point at 151.8°C. Its enthalpy (ΔHf) was 266.5 J/g ( and ). PZA displayed an endothermic peak at 187.3°C, a melting point at 182.7°C and ΔHf at 200.5 J/g ().
Table 2. Differential scanning calorimeter data of porous mannitol, PZA-proliposome formulations and PZA.
Figure 3. Differential scanning calorimeter thermogram of (A) porous mannitol, (B–F) PZA-proliposome formulation I to V (containing PZA 10, 20, 40, 60 and 80% w/w, respectively) and (G) PZA.
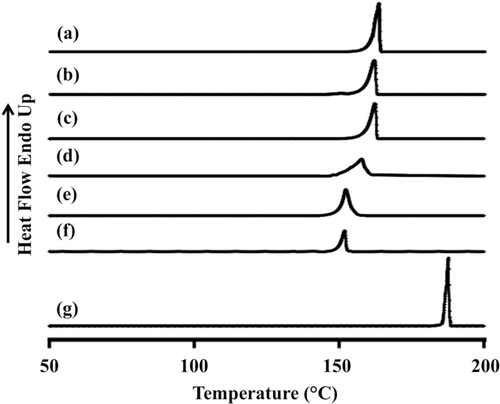
The endothermic peak, melting point and ΔHf of PZA-proliposome formulation I to V are shown in – and . They were less than that of porous mannitol and PZA. They tended to decrease when the PZA ratio increased. The DSC curves of PZA-proliposome formulations III to V that showed a broadening of the endothermic peak and shifts to a lower temperature could indicate that there was some interaction between PZA itself or with the porous mannitol. These results also correlated with the X-ray diffraction patterns of PZA-proliposome formulation II, III, IV and V. This point will be discussed in the next section.
Fourier transform-infrared spectroscopy of PZA-proliposome
To investigate the relationships between the components of the proliposome, FT-IR studies were conducted. It is well known that the hydroxyl group of the sugar may interact with the amine group of drugs (Ross and Rekharsky, Citation1996). Therefore PZA may interact with mannitol or with PZA itself. It was observed that there were changes in the FT-IR spectra in the absorption bands of the amine groups and hydroxyl groups.
The mannitol spectrum () showed characteristic bands of hydroxyl in the plane vibration (1419 cm−1) and hydroxyl stretching vibration (1080 and 1019 cm−1). The characteristic peaks for pyrazinamide () was seen at 1716 cm−1, 1610 cm−1 and 1580 cm−1 corresponding to amide I (C = O stretching vibration), amine bending and amide II (N-C = O symmetric stretching vibration), respectively. In the spectrum of the formulations of I to V (–), the amine peak shifted from 1610 cm−1 to 1637 cm−1 for formulation I and II, to 1638 cm−1 for formulation III and IV and to 1672 cm−1 for formulation V, respectively. The peak of amide I (1716 cm−1) and amide II (1580 cm−1) were absent. These results indicated that some function groups were altered.
Figure 4. FT-IR spectra of (A) porous mannitol, (B–F) PZA-proliposome formulation I to V (containing PZA 10, 20, 40, 60 and 80% w/w, respectively) and (G) PZA.
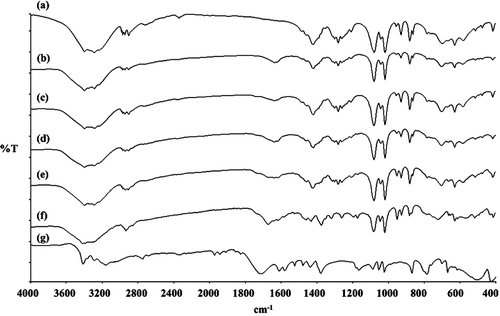
From the X-ray diffraction patterns, DSC data and FT-IR spectra, all data indicated that there was some interaction in PZA-proliposomes especially in formulations II to V. It could be explained that there might be an interaction between PZA and mannitol or PZA itself that occurred during the spray drying process. PZA containing an amide group and mannitol - an alcoholic sugar, they might interact/form hydrogen bonding between the carbonyl of the amide and the hydroxyl group and PZA can interact with PZA (intermolecular bonding) through hydrogen bonding of the carbonyl in the amide and hydrogen in primary amine.
Cytotoxicity of PZA-proliposome to cells in the respiratory tract
Human bronchial epithelial cells (Calu-3) were used in this study as an upper airway cell. The human lung adenocarcinoma cells (A549) are human alveolar basal epithelial cell, were representation of lower airway cells. AMs (NR8383) were used as a target site for anti-TB delivery.
The viabilities of Calu-3, A549 and NR8383 were determined after being challenged with different concentrations of PZA and PZA-proliposomes (Formulation I and V). Formulations I and V were the selected formulations because their porous mannitol content varied from the highest to the lowest. The effect of PZA, present with different amounts of the porous mannitol in the formulations was established on the respiratory cell lines.
Calu-3 cell viability after being exposed to PZA, PZA-proliposome formulation I and V at various concentrations was less than 80%, while the cell viability was more than 80% only in the case of exposure to PZA-proliposome formulation I concentration of less than 50 µg/ml (). At concentrations of 25–500 µg/ml of PZA these were toxic to A549 cells (). PZA-proliposome formulation I and V at less than 250 µg/ml were not toxic to A549 cells (cell viability of more than 80%). For NR8383 cell viability, after being exposed to PZA, was less than 80% (). PZA-proliposome formulation I and V were not toxic to NR8383 cells when the concentration was 25–500 µg/ml.
Figure 5. Viability of Calu-3 (A), A549 (B) and NR8383 (C) cell lines after exposed with different concentrations of PZA (•), PZA-proliposome formulation I (▴) and PZA-proliposome formulation V (▪) (mean ± SD, n ≥ 6).
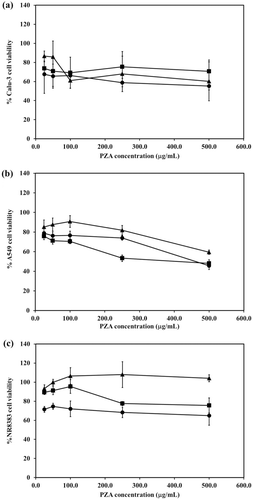
In all cells, the cytotoxicity results were similar. Cell viability after being exposed to PZA-proliposome formulation I and V were higher than for PZA. These results indicated that proliposome could be beneficial to reduce drug toxicity to cells in the respiratory tract.
Effect of PZA-proliposome on production of IL-1β, TNF-α and nitric oxide (NO) by NR8383 cell line
The amount of IL-1β generated by NR8383 cells in response to PZA-proliposome and PZA, compared to that to the lipopolysaccharide (LPS) from E. coli was minute (). LPS at 31.25 ng/ml stimulated NR8383 cells to produce 173 ± 47.4 pg/ml IL-1β and with 500 ng/ml of LPS, NR8383 cells produced 419 ± 31.8 pg/ml IL-1β. After challenge with PZA-proliposome and PZA containing PZA at concentrations of between 25–500 µg/ml, IL-1β was produced by NR8383 cells at less than 5 pg/ml. No significant differences in IL-1β concentrations were observed after NR8383 cells were challenged with PZA-proliposome and PZA containing PZA less than 125 µg/ml. After challenge with PZA itself and PZA-proliposome formulation V and formulation I containing 500 µg/ml PZA, NR8383 cells produced 2.5 ± 1.2, 1.0 ± 0.3 and 0.6 ± 0.1 pg/ml IL-1β respectively. These results demonstrated that PZA-proliposome could be used as a delivery system without generating a significant production of IL-1β from AMs.
Figure 6. The level of inflammatory cytokine (IL-1β (A) and TNF-α (B)) and nitric oxide (C) produced from NR8383 cell lines after exposure with different concentrations of PZA (•), PZA-proliposome formulation I (▴), PZA-proliposome formulation V (▪) and LPS from E. coli (○) for 24 h (mean ± SD, n ≥ 6).
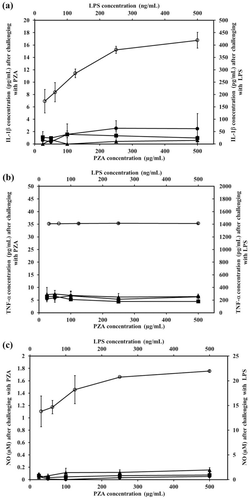
From , LPS (31.25–500 ng/ml) stimulated NR8383 cells to produce 1385–1425 pg/ml TNF-α while after stimulation by PZA in the range of 125–500 µg/ml present in PZA-proliposome and PZA itself the TNF-α generated from NR8383 was less than 10 pg/ml. Increasing the amount of PZA in the PZA-proliposomes and PZA over the range of 31.25–500 µg/ml had no effect on the amount of TNF-α generated from NR8383 cells (p > 0.05).
NO produced from NR8383 cells responding to PZA-proliposome and PZA, compared to the NO response to LPS is shown in . The response was similar to that observed for IL-1β and TNF-α production. LPS at 31.25 and 500 ng/ml stimulated NR8383 cells to produce NO 13.8 ± 3.1 and 22.0 ± 0.2 µM, respectively. NO generated from NR8383 cells responding to PZA-proliposome and PZA containing between 31.25–500 µg/ml PZA was less than 0.2 µM.
As expected, NR8383 cells produced lower amounts of IL-1β, TNF-α and NO in response to PZA-proliposome and PZA, compared to LPS. The concentration of LPS used to stimulate NR8383 cells to produce an immunological response was 1000 times less than the concentration of any amount of PZA administered as PZA itself or in PZA-proliposomes. LPS activation of NR8383 cells to produce inflammatory cytokines was significantly greater than when it was challenged with PZA-proliposomes (p < 0.05).
Phagocytosis of anti-TB dry powder particles by macrophage cells
NR8383 cells were bright green cells when the cell photographs were taken in bright field mode. To observe the phagocytosis, PZA-proliposome formulation I was reconstituted in distilled water and stained with Lumidot® 640 because Lumidot® 640 could be read in the fluorescence mode. shows phagocytosis of NR8383 cells taken in different modes. In the overlay mode, the intensity of the red color increased. The phagocytosis occurred within 2 min as there was no difference between data of 2 min and 30 min. The phagocytosis was observed not only by the increase in the intensity of the red, but NR8383 cells were larger and some of them changed their shapes. It indicated that NR8383 could phagocytose the particles stained with Lumidot® 640. These results also correlated with the size measurement after the reconstitution study. Kanchan and Panda (Citation2007) reported that nanoparticles of 200–600 nm in size were efficiently phagocyted. These results supported the observation that PZA-liposomes obtained from a PZA-proliposome reconstitution could be phagocyted by NR8383 cells.
An in vivo repeated dose toxicity study of the proliposomes
From the minimum inhibitory concentration of PZA for susceptible of M. tuberculosis, which were determined using radiometric techniques, are 6.2–50 µg/ml when tested at pH of 5.5 (Salfinger and Heifets, Citation1988). The expected PZA amount in lung was at least 20 µg/kg body weight. Therefore PZA-proliposome formulation I was inhaled at least 200 µg/kg body weight. PZA-proliposome formulation I amount was calculated to be 4 mg/kg body weight for inhalation (20 times of expected PZA amount in lung). The toxicity studies were carried out at these extremely high doses for practical reasons associated with real life application because only a fraction of dose delivery reached alveoli. For the repeated dose toxicity experiment, the samples were administered by intratracheal instillation at day 0 and administered daily by intratracheal inhalation until day 28. During this experimental period, no significant abnormalities in food intake, feces, hair and behaviour in any group of animals were observed. Animals in all groups gained weight over the duration of the study and no statistically significant changes were observed when compared with the control group (p > 0.01). For the serum biochemical parameters, a significant increase in AST was observed in all groups after administration for 7 days. Apart from that, there were no significant differences in BUN, Cr and ALT (). The ALT data showed a tendency to increase. The increase in AST might result from the animals readjusting themselves to the new environment. Although AST values increased slightly, they were still within the normal biochemical ranges for rats (52–224 U/l) (Sharp and LaRegina, Citation1998). Serum levels for both ALT and AST become elevated whenever disease processes affect liver cells, with ALT being the more liver-specific enzyme. Elevations of ALT activity persisted longer than those of the AST activity (Johnston, Citation1999). In this study, renal and liver toxicity in the repeated dose toxicity study were not observed.
Conclusions
PZA-proliposome powders were successfully produced by spray drying. Depending on the ratios of PZA to mannitol, the powders reflected the crystalline nature of the respective components and the varying degrees of interaction with the drug present in the formulations. Dispersion of the powders showed MMADs in a range of 4.26–4.39 μm, with a FPF of 20–30%. PZA and PZA-proliposomes were shown to be less toxic to respiratory-associated cells, and also did not activate AM to produce inflammatory cytokines and nitric oxide at a level that would cascade to a secondary inflammation. In vivo repeated dose toxicity study, all animal survived throughout 28 days without renal and liver toxicity.
Declaration of interest
This work was supported by National Research Council of Thailand and Prince of Songkla University National Research University Project of Thailand’s Office of the Higher Education Commission. The authors report no declarations of interest.
References
- Agnihotri SA, Soppimath KS, Betageri GV. 2010. Controlled release application of multilamellar vesicles: a novel drug delivery approach. Drug Deliv 17:92–101.
- Ahsan F, Rivas IP, Khan MA, Torres Suarez AI. 2002. Targeting to macrophages: role of physicochemical properties of particulate carriers – liposomes and microspheres – on the phagocytosis by macrophages. J Control Release 79:29–40.
- Alving CR. 1998. Macrophages as targets for delivery of liposome-encapsulated antimicrobial agents. Adv Drug Deliv Rev 2:107–128.
- Atkins PJ, Barker NP, Mathisen D. 1992. The design and development of inhalation drug delivery systems. In: Pharmaceutical Inhalation Aerosol Technology. Hickey AJ (Ed.). Marcel Dekker: New York, USA.
- Brain JD, Knudson DE, Sorokin SP, Davis MA. 1976. Pulmonary distribution of particles given by intratracheal instillation or by aerosol inhalation. Environ Res 11:13–33.
- Castro RAE, Teresa MRM, Evora AOL, Feiteira JC, Silva MR, Beja AM, Canotilho J, Eusebio MES. 2010. A new insight into pyrazinamide polymorphic forms and their thermodynamic relationships. Cryst Growth Des 10:274–282.
- Changsan N, Chan HK, Separovic F, Srichana T. 2009a. Physicochemical characterization and stability of rifampicin liposome dry powder formulations for inhalation. J Pharm Sci 98:628–639.
- Changsan N, Nilkaeo A, Pungrassami P, Srichana T. 2009b. Monitoring safety of liposomes containing rifampicin on respiratory cell lines and in vitro efficacy against Mycobacterium bovis in alveolar macrophages. J Drug Target 17:751–762.
- Crowder TM, Rosati JA, Schroeter JD, Hickey AJ, Martonen TB. 2002. Fundamental effects of particle morphology on lung delivery: predictions of Stokes’ law and the particular relevance to dry powder inhaler formulation and development. Pharm Res 19:239–245.
- Elhissi AM, Faizi M, Naji WF, Gill HS, Taylor KM. 2007. Physical stability and aerosol properties of liposomes delivered using an air-jet nebulizer and a novel micropump device with large mesh apertures. Int J Pharm 334:62–70.
- Elhissi AMA, Islam MA, Arafat B, Taylor M, Ahmed W. 2010. Development and characterisation of freeze-dried liposomes containing two anti-asthma drugs. Micro & Nano Letters 5:184–188.
- Heurtault B, Saulnier P, Pech B, Proust JE, Benoit JP. 2003. Physico-chemical stability of colloidal lipid particles. Biomaterials 24:4283–4300.
- Thompson DC. 1992. Pharmacology of therapeutic aerosols. In: Pharmaceutical Inhalation Aerosol Technology. Hickey AJ (Ed.). Marcel Dekker: New York, USA.
- Huttunen K, Ruotsalainen M, Iivanainen E, Torkko P, Katila M, Hirvonen M. 2000. Inflammatory responses in RAW264.7 macrophages caused by mycobacteria isolated from moldy houses. Environ Toxicol Pharmacol 8:237–244.
- Johnston DE. 1999. Special considerations in interpreting liver function tests. Am Fam Physician 59:2223–2230.
- Kanchan V, Panda AK. 2007. Interactions of antigen-loaded polylactide particles with macrophages and their correlation with the immune response. Biomaterials 28:5344–5357.
- Khuller GK, Kapur M, Sharma S. 2004. Liposome technology for drug delivery against mycobacterial infections. Curr Pharm Des 10:3263–3274.
- Mitchison DA, Fourie PB. 2010. The near future: improving the activity of rifamycins and pyrazinamide. Tuberculosis (Edinb) 90:177–181.
- Morris KR, Rodriguez-Hornedo N. 1993. Hydrates. In: Encyclopedia of Pharmaceutical Technology. Swarbrick J, Boylan JC (Eds.). Marcel Dekker: New York, USA. 393–440.
- Nolan LM, Tajber L, McDonald BF, Barham AS, Corrigan OI, Healy AM. 2009. Excipient-free nanoporous microparticles of budesonide for pulmonary delivery. Eur J Pharm Sci 37:593–602.
- Pandey R, Sharma S, Khuller GK. 2004. Nebulization of liposome encapsulated antitubercular drugs in guinea pigs. Int J Antimicrob Agents 24:93–94.
- Punturee K, Wild CP, Vinitketkumneun U. 2004. Thai medicinal plants modulate nitric oxide and tumor necrosis factor-alpha in J774.2 mouse macrophages. J Ethnopharmacol 95:183–189.
- Rojanarat W, Changsan N, Tawithong E, Pinsuwan S, Chan HK, Srichana T. 2011. Isoniazid proliposome powders for inhalation-preparation, characterization and cell culture studies. Int J Mol Sci 12:4414–4434.
- Ross PD, Rekharsky MV. 1996. Thermodynamics of hydrogen bond and hydrophobic interactions in cyclodextrin complexes. Biophys J 71:2144–2154.
- Saetern AM, Flaten GE, Brandl M. 2004. A method to determine the incorporation capacity of camptothecin in liposomes. AAPS PharmSciTech [Online] 5:1–8. http://www.aapspharmscitech.org. Accessed on 14 September 2010.
- Salfinger M, Heifets LB. 1988. Determination of pyrazinamide MICs for Mycobacterium tuberculosis at different pHs by the radiometric method. Antimicrob Agents Chemother 32:1002–1004.
- Sharp PE, LaRegina MC. 1998. The laboratory rat. In: The Laboratory Animal Pocket Reference Series. Suckow MA (Ed.). CRC Press LLC: Florida, USA. 17.
- Srichana T, Suedee R, Srisudjai P. 2003. Application of spectrofluorometry for evaluation of dry powder inhalers in vitro. Pharmazie 58:125–129.
- Taylor K, Elhissi A. 2006. Preparation of liposomes for pulmonary delivery using medical nebulizers. In: Liposome Technology. Kevin M, Tayler G, Abdelbary M, Elhissi A (Eds.). Informa Healthcare: London, England. 67–84.
- USP 30 and NF 25; USP-NF. 2007. Guideline No. 905: Uniformity of dosage units. In: US Pharmacopeia 30-NF 25. US Pharmacopeial Convention (ed.). US Pharmacopeial Convention, Inc.: Rockville, USA. 378–384.
- Wagner A, Vorauer-Uhl K. 2011. Liposome technology for industrial purposes. J Drug Deliver. doi:10.1155/2011/591325. http://www.hindawi.com. Accessed on 11 July 2011.