Abstract
Context: Delivery of drugs from the nasal cavity to the brain is becoming more widely accepted, due to the non-invasive nature of this route and the ability to circumvent the blood brain barrier (BBB).
Objective: Because of similarities in the proteins comprising the olfactory epithelial tight junction (TJ) proteins and those of the BBB, we sought to determine whether papaverine (PV), which is known to reversibly enhance BBB permeability, could increase the delivery of intranasally administered gemcitabine to the central nervous system in rats.
Experimental methods: Included intranasal administration of gemcitabine, fluorescein isothiocyanate-dextran beads and PV, histopathology, immunostaining, RT-PCR, western blot analysis, immunofluorescence localization, spectrofluorometric analysis, in vivo brain microdialysis, HPLC analysis and in vitro gemcitabine recovery.
Results and discussion: PV transiently decreased the levels and altered immunolocalization of the TJ protein phosphorylated-occludin in the olfactory epithelium, while causing an approximately four-fold increase in gemcitabine concentration reaching the brain. The enhanced delivery was not accompanied by nasal epithelial damage or toxicity to distant organs.
Conclusions: The ability to transiently and safely increase drug delivery from the nose to the brain represents a non-invasive way to improve treatment of patients with brain disorders.
Introduction
Delivery of therapeutics to the brain to treat neurological diseases and other central nervous system (CNS)-related disorders is a challenge due to the impenetrable nature of the blood brain barrier (BBB). Nucleoside drugs are important tools in treating many important diseases, including HIV/AIDS and various cancers (Davis et al., Citation2001; Wong et al., Citation2007; Schifitto et al., Citation2007). While oral or injection treatments with these drugs make them systemically bioavailable, they do not penetrate efficiently from the bloodstream into the brain due to the presence of BBB.
The BBB prevents the net movement of polar and high molecular weight molecules from the bloodstream into the brain using a combination of efflux transporters and tight junction (TJ) proteins on endothelial cells (Ge et al., Citation2005; Hawkins & Davis, Citation2005; Hawkins & Egleton, Citation2006; Persidsky et al., Citation2006). The proteins involved in forming BBB TJs include claudins, occludin, junctional adhesion molecules, and cytoplasmic proteins such as zona occludens (Furuse et al., Citation1993; Wolburg & Lippoldt, Citation2002; Persidsky et al., Citation2006; Ueno, Citation2007; Stamatovic et al., Citation2008). Down regulation of TJ proteins increases the permeability of the BBB (Wolburg & Lippoldt, Citation2002; Argaw et al., Citation2009). Studies have shown BBB disruption using ionizing radiation and vasoactive drugs can cause transient increase in uptake of chemotherapeutic drugs to the brain (Griffin et al., Citation1977; Levin et al., Citation1979; Olesen & Crone, Citation1986). Increased permeability of the entire BBB, while potentially beneficial for drug delivery purposes, can have adverse effects, including the potential for brain edema and the passage of infectious agents from the bloodstream into the brain.
The intranasal (IN) route is becoming more widely used for administration of pharmaceutical agents and vaccines. The pathways along the olfactory and trigeminal nerves that innervate the nasal passages are involved in the nasal uptake of toxicants and drugs by transneuronal or paracellular transport (Frey, Citation2002; Thorne et al., Citation2004; Dhanda et al., Citation2005; Pardridge, Citation2005). The benefits of IN administration include greater acceptance by patients, particularly children, and the access that the IN route provides to the CNS, thus avoiding first pass clearance by the liver and potential toxicity to non-target organs. Recent successes include use of IN-administered midazolam for the treatment of status epilepticus in children and young adults (McMullan et al., Citation2010); IN scopolamine for motion sickness (Simmons et al., Citation2010), and IN insulin has been shown to improve cognition in early Alzheimer’s disease (Benedict et al., Citation2004).
Numerous investigators have investigated various strategies to enhance IN drug delivery to the CNS. Many of these strategies resulted in severe, and often irreversible, damage to the olfactory mucosa (Davis & Illum, Citation2003). Therefore, we decided that we would attempt to enhance intranasally administered nucleoside drug delivery to the brain by transiently increasing paracellular transport between cells of the olfactory mucosa. Olfactory epithelial TJs express many proteins also localized to the BBB, including zona occludens 1 (ZO-1), occludin, and claudin-5 (CLDN5) (Wolburg et al., Citation2008). Because of the similarity in structure between the BBB and olfactory epithelial TJs, we propose that a strategy that has been demonstrated to be effective in transiently increasing permeability of the BBB will also transiently perturb olfactory epithelial TJs, thereby enhancing the delivery of IN-administered drugs to the CNS.
Papaverine (PV) is a potent vasodilator acting as a phosphodiesterase (PDE) inhibitor and is used in human medicine for indications ranging from treatment of cerebral vascular constrictions to treatment of erectile dysfunction (Mooradian et al., Citation1989; Kaku et al., Citation1992; Fandino et al., Citation1998; Schuknecht et al., Citation1999; Minami et al., Citation2001). PV is a specific inhibitor of PDE10A, which is localized in the striatum, testis, epididymal sperm, and enteric ganglia (Fujishige et al., Citation1999a,Citationb; Coskran et al., Citation2006). PV has also been shown to transiently down regulate BBB proteins in rats (Bhattacharjee et al., Citation2001; Platz et al., Citation2008; Wang et al., Citation2010). When administered via the external carotid artery, PV (0.2%) caused an increase in cerebrovascular permeability to 14C-sucrose lasting ∼3 h (Bhattacharjee et al., Citation2001). Similarly, intracarotid infusion of 0.15% PV caused increased blood-tumor barrier (BTB) permeability lasting approximately 2 h, with down regulation of occludin, CLDN5 and F-actin (Wang et al., Citation2010). We hypothesized that IN-administered PV would also safely and transiently perturb nasal epithelial TJs.
In the present study, we first characterized the effects of PV on the olfactory epithelium (OE) using histological, western blot and RT-PCR techniques. After confirming that our IN drug delivery technique targeted the OE without toxicity to target and non-target tissues, we then used in vivo brain microdialysis to investigate the potential for PV to enhance delivery of IN-administered gemcitabine, a model nucleoside chemotherapeutic drug (Santoro et al., Citation2000; De Lange et al., Citation2004), to the CNS. Gemcitabine, our model drug, is a small (MW 299.66) polar molecule and has 7% plasma protein binding ratios in rat and dog and 10% in human (Esumi et al., Citation1994). Since it is a small hydrophilic molecule, it can be transported across the nasal epithelial TJs by paracellular transport. It has shown to be effective in the treatment of some solid tumors (Santoro et al., Citation2000; De Lange et al., Citation2004). A previous study has demonstrated increased gemcitabine uptake into brain tumors as compared to normal brain tissue, due to differential permeability of tumors (Apparaju et al., Citation2008; Sigmond et al., Citation2009). We hypothesize that increasing the permeability of nasal epithelial TJs using PV will enhance the delivery of gemcitabine from the nasal cavity to the CNS. One challenge of this project was to understand whether PV’s activity on olfactory epithelial TJ is related to its PDE activity, or its transient effect on TJ proteins. The results of our study could improve the outcome for individuals affected by CNS-associated disorders by improving drug delivery via localized enhancement across the nasal epithelia.
Materials and methods
Chemicals and supplies
Gemcitabine (2′,2′-difluorodeoxycytidine) hydrochloride was obtained from Enzo Life Sciences (Farmingdale, NY). The drug was reconstituted for IN administration in sterile 0.9% sodium chloride to a final concentration of 150 mg/mL. PV hydrochloride was obtained from Fisher Scientific (Pittsburgh, PA). Tetrahydrouridine, a deoxycytidine deaminase inhibitor, was obtained from Calbiochem (San Diego, CA). Cannulation materials for the implantation were purchased from Plastics one Inc. (Roanoke, VA) and VWR Scientific (Batavia, IL). Dialysis membrane with a 13 000 MW cutoff and an outside diameter of 210 µm was purchased from Spectrum Laboratories (Rancho Dominguez, CA). HPLC grade solvents were obtained from Fisher Scientific and VWR International (Arlington Heights, IL). Concentric-style microdialysis probes were constructed as described (Yamamoto & Pehek, Citation1990). Fluorescein isothiocyanate-dextran beads (FD4, average molecular weight 3000–5000) were obtained from Sigma Aldrich (St. Louis, MO) for the IN drug distribution study.
Animals
Male Sprague-Dawley rats (220–250 g) were obtained from Harlan, Indianapolis, IN. The animals were housed individually in a room temperature maintained at 72 °F, 55% relative humidity with a 12-h/12-h light/dark cycle, and provided access to standard laboratory chow and tap water ad libitum. All procedures adhered to the “Principles of Laboratory Animal Care” guidelines and were approved by the University of Cincinnati Institutional Animal Care and Use Committee.
Histology
In order to determine whether PV treatment caused damage to nasal epithelia, PV was administered for five consecutive days (0.3, 0.8, or 1.4% in left nostril; 60 μL/nostril) and rats were sacrificed (carbon dioxide asphyxiation) for histological examination. PV doses were selected on the basis of previous studies showing transient increases in BBB and BTB permeability (Yoshimura et al., Citation1996; Sawada et al., Citation1997; Bhattacharjee et al., Citation2001; Platz et al., Citation2008; Wang et al., Citation2010). Nasal cavities were fixed in 10% neutral buffered formalin and decalcified in 10% formic acid prior to paraffin embedding. Sections (5 µm in thickness), taken at standard levels of the nasal cavity (Young, 1981), were stained with hematoxylin and eosin (H&E). Another group of animals was treated with a single dose of PV (1.4%; 60 μL in left nostril) and sacrificed 1 d or 5 d later for examination of other tissues for potential toxicity induced by PV. Nasal cavities, liver, kidney, spleen and lungs were collected, fixed in formalin, and embedded in paraffin. Lungs were fixed by inflation with formalin (30 cm height). Sections (5 µm) were stained with H&E and examined by light microscopy.
Immunohistochemistry
Olfactory marker protein (OMP) is a marker of mature olfactory neurons and their axons. OMP immunohistochemistry (antibody was the generous gift of Dr Frank Margolis, University of Maryland) was conducted as described previously (Genter et al., 2000) to further confirm that PV treatment did not damage olfactory neurons. Immunolocalization was detected using peroxidase Goat IgG vectastain ABC kit (Vector Labs, Burlingame, CA). Immunostained sections were counterstained with hematoxylin and digitized with a Nikon D80 camera.
RNA isolation, cDNA synthesis and PDE10A RT-PCR
Rats were sacrificed by decapitation. Striatum, OE and olfactory bulb (OB) were collected from untreated control rats, immediately frozen on dry ice and stored at −80 °C until RNA isolation. RNA was isolated using Tri Reagent (Molecular Research Center, Cincinnati, OH) according to the manufacturers’ protocol. Total RNA quantity and quality were assessed by absorbance (260 nm) and absorbance ratio of 260/280 nm utilizing Agilent Bioanalyzer/Nanodrop analysis (Agilent 2100 Bioanalyzer, Santaclara, CA). RNA samples with absorbance(260/280) ratios 1.95–2.0 were used for qRT-PCR. cDNA synthesis was performed using an RNA-to-cDNA kit (Verso cDNA synthesis kit, #AB1453, Thermo Fisher Scientific, Waltham, MA). The following PCR primers for rat PDE10A gene were used: sense 5′-TGCTTCCGAAGGCTGACCGA-3′ and antisense 5′-GCACTCTCCAAGGAAGTACA-3′, which amplified a 360-bp fragment (Giorgi et al., Citation2011). Forty PCR cycles were performed on cDNA after an initial denaturing step of 4 min at 94 °C using the following profile: 94 °C for 1 min; 60 °C for 2 min; 72 °C for 3 min, including a final extension of 7 min at 72 °C. The PCR products were separated by electrophoresis on a 2% agarose gel and detected with ethidium bromide staining.
Western blot analysis
Western blot analysis was performed to investigate the expressions of occludin and claudin-5 at different time points after PV administration. Rats were treated with 1.4% PV (60 μL in each nostril) and sacrificed 0, 15, 30, 60 and 120 min after PV treatment. OE from the ethmoid turbinates (ET) and caudal one-third of the nasal septum was immediately frozen on dry ice, and stored at −80 °C. Tissues were homogenized in protein lysis buffer (0.1 mM PMSF, 50 mM Tris HCl; pH 7.6, 2 M urea, 0.1% NaCl, 0.1% EDTA and 0.1% Brij 35), centrifuged at 31 360 g (4 °C) for 15 min and supernatant was collected. The Bradford assay was used to measure the protein concentration in collected supernatants. Equal amounts of protein (20 μg) were separated by SDS-PAGE and transferred to nitrocellulose for immunoblotting with antibodies for occludin (1.25 μg/mL, Invitrogen, Carlsbad, CA) and claudin-5 (2.5 μg/mL, Invitrogen). Membranes were stained with Ponceau Red (Sigma, St. Louis, MO) to assure equal protein loading. β-Actin (diluted 1:10 000, Santa Cruz Biotechnology, Inc., Santa Cruz, CA) was used as an internal control. Immune complexes were visualized using the enhanced chemiluminescence (Pierce ECL western blotting substrate, Thermo Scientific, Waltham, MA). The protein bands were scanned using Epson perfection V750 Pro scanner, and band densities were calculated by ImageJ software (http://rsbweb.nih.gov/ij/) and normalized with that of β-actin.
Immunofluorescence localization of occludin following PV treatment
In order to determine whether PV treatment alters the immunolocalization of the nasal epithelial TJ protein occludin, PV was administered IN (1.4% PV; 60 μL/nostril) and rats were sacrificed (carbon dioxide asphyxiation) after 0, 15, 30, 60 and 120 min. Nasal cavities were fixed in 10% neutral buffered formalin and decalcified in 10% formic acid prior to paraffin embedding. Sections (5 μm in thickness) were taken at standard levels of the nasal cavity (Young, 1981). Anti-occludin antibody (1:400, Santa Cruz Biotechnology Inc.) was incubated with the nasal section slides overnight at 4 °C. The slides were then incubated with secondary antibody Alexa Fluor 488 Donkey anti-rabbit IgG (1:200, Invitrogen) for 2 h at room temperature in dark. The slides were mounted with a coverslip using Vectashield HardSet Mounting Medium with DAPI (Vector; H-1500, Burlingame, CA). Nasal sections were photographed individually with a Leica TCS SP Spectral Confocal microscope.
IN drug distribution study
FD4 beads were used to determine IN drug distribution. Rats were lightly anesthetized with ketamine/xylazine (87/13 mg/kg i.p.) and FD4 was administered intranasally (60 μL/nostril; 600 μg/animal, n = 4) using sterile micropipette tips. Four hours after IN FD4, animals were sacrificed. The following tissues were collected and stored at −80 °C for fluorescence analysis: OE was collected from the ET; respiratory epithelium (RE) was collected from the maxilloturbinates and nasoturbinates; OB, forebrain (FB), hindbrain (HB) and cerebellum (CE). Tissues were homogenized in protein lysis buffer (0.1 mM PMSF, 50 mM Tris HCl; pH 7.6, 2 M urea, 0.1% NaCl, 0.1% EDTA and 0.1% Brij 35), centrifuged at 31 360 g (4 °C) for 15 min and supernatant was collected. The Bradford assay was used to measure the protein concentration in collected supernatants.
Spectrofluorometric analysis
The supernatants were heated for 10 min at 70 °C and centrifuged at 20 070 g for 10 min to remove heme, as heme has the same excitation and emission wavelength as FD4. Clear supernatant was collected, and fluorescence was measured using a Hitachi F-4500 spectrofluorometer (EX = 493 nm, EM = 518 nm, slit width – 5, 20). In order to correct for potential tissue auto fluorescence, the corresponding tissues were assayed from rats not treated with FD4. Values obtained from the untreated animals, while negligible, were subtracted from the fluorescence values for the FD4-treated rats.
In vivo brain microdialysis cannulation procedure
Rats were anesthetized with an injection of ketamine and xylazine (87/13 mg/kg, i.p.). The coordinates for the striatum of the brain were identified according to the atlas of Paxinos and Watson (1.2 mm anteroposterior and ±3.1 mm lateral from bregma) and a hole was drilled through the cranium, dorsal to the striatum (Paxinos & Watson, Citation2006). A guide cannula was then lowered into the hole and secured to the skull with screws and cranioplastic cement. Animals were allowed to recover for 3 d before the drug administration and sampling.
PV administration and sampling protocol
On the morning of the experiment, concentric style microdialysis probes were inserted through the guide cannulae. The length of the dialysis membrane for the striatum was 2.5 mm. The probes were perfused with simulated brain interstitial fluid (Dulbecco’s phosphate-buffered saline containing 1.2 mM CaCl2 and 5 mM glucose) at an input rate of 1.8 µL/min, in order to facilitate an optimal temporal resolution and higher recovery of gemcitabine. Probes were perfused and allowed to equilibrate for 1.5 h before initiating drug administration and sampling procedures.
Before administering drugs intranasally, the rats were anesthetized with ketamine/xylazine. PV solutions [3 mg/mL (0.3%), 8 mg/mL (0.8%) and 14 mg/mL (1.4%)] were prepared in 0.9% saline. PV was administered intranasally at 60 µL/nostril in each nostril and 15 min after PV treatment; gemcitabine (50 mg/kg; also dissolved in sterile saline) was administered intranasally (n = 4/treatment group). Gemcitabine dose was selected on the basis of previous studies (Degen et al., Citation2003; Apparaju et al., Citation2008). A control group of animals (n = 4) received saline infusion instead of PV, followed by gemcitabine.
Dialysate samples were collected in tubes containing the cytidine deaminase inhibitor tetrahydrouridine (0.5 mM final concentration) every 30 min after gemcitabine treatment for 8 h.
HPLC analysis of gemcitabine in dialysate
The analysis of gemcitabine was done using a slight modification of a published procedure (Shipley et al., Citation1992). The mobile phase consisted of ammonium acetate:acetonitrile (98:2, v/v) buffer (0.1 M) [with acetic acid (0.05%) and triethylamine to adjust the pH to 7.0]. The mobile phase was pumped at a flow rate of 1 mL/min using a Waters 510 pump. Gemcitabine was eluted on a Waters Nova-Pak® C18 4 µm column coupled with a guard column of similar properties. A Waters 486 variable wavelength UV/VIS detector coupled to millennium software was used for detecting and integrating the sample peaks. The lower limit of quantitation for gemcitabine using this method of analysis was 25 ng/mL for the microdialysate samples. The intra- and inter-day precision of the analyses was <15%. The accuracy was within ±12%.
Gemcitabine concentration in brain extracellular fluid
Non-compartmental analysis of gemcitabine concentration versus time data in brain extracellular fluid (BECF) was performed employing WinNonlin version 6.1 (Pharsight, CA). The BECF concentrations of gemcitabine were time-averaged over the dialysate collection interval. The pharmacokinetic parameter, area under the curve (AUC) values of gemcitabine in BECF was calculated employing the linear trapezoidal rule, where the total area under the drug concentration versus time curve was measured by summing up a number of incremental areas from each trapezoid.
where Ci and Ci+1 are the concentrations at time points ti and ti+1, respectively, and the time interval, Δt = (ti+1 – ti).
Since the sampling interval of 30 min employed in our study may not facilitate temporal resolution required for precise determination of elimination half-life, only AUC0–t, where t is the time corresponding to the last sample collection, was determined.
In vitro recovery
In order to relate the concentrations in the dialysate to the true concentrations in the tissue outside the probe and to measure the extent to which the compound of interest is recovered by the dialysate, in vitro recovery was done. Recovery was determined with the probe in aqueous solutions containing 20, 50 or 63.3 mM gemcitabine. The probe was then continuously perfused at a constant flow rate with dialysis fluid (Dulbecco’s phosphate-buffered saline containing 1.2 mM CaCl2 and 5 mM glucose) devoid of gemcitabine, and samples were collected during fixed time intervals. The percent recovery of the solute was then determined as follows:
where Cdial is the concentration of gemcitabine in the dialysate sample and Cbulk is the concentration of gemcitabine in the bulk reservoir.
Statistical analysis
Analysis of variance (ANOVA) was used to measure the statistical differences. Multiple comparisons were carried out using Tukey’s honest significant difference method.
Results
Response to IN PV
PV treatment (once/d for 5 d) caused no histological damage to the OE (). IN PV at concentrations of up to 1.4% for up to 5 d did not affect OMP immunostaining (). Lung, liver, kidney and spleen from PV (1.4%)-treated rats showed no histological damage (Supplemental Figure 1).
Figure 1. Lack of nasal toxicity: (a) Frontal nasal cavity section showing no damage to nasal epithelia comparing the 1.4% PV-treated (**) and untreated sides of the nose. NS = nasal septum. (b) OMP staining, showing normal staining in the treated side of nasal cavity (*) of a rat treated with 0.8% PV (left) and in the OB (right) corresponding to the treated side of the nasal cavity. High power photomicrograph (middle) shows normal cellular structure and OMP immunoreactivity in the olfactory epithelium (OE) and in subepithelial nerve bundles (NB).
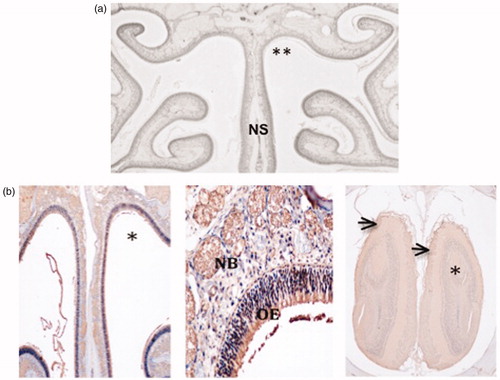
PDE10A expression using RT-PCR
Sharp bands were observed in striatum (positive control) and OB indicating PDE10A expression in these tissues. No band was observed in OE, suggesting that PDE10A is not expressed in OE (data not shown).
Response of nasal epithelial TJ proteins to IN PV treatment
Fifteen minutes after IN PV treatment (1.4%, 60 μL/nostril), phosphorylated occludin immunoreactivity in OE decreased. The lowest level appeared at 30 min and increased thereafter (). For phosphorylated occludin, the different time points showed significant differences (p < 0.05). There was no significant change in the expression levels of CLDN5 at any time point (p = 0.59) (data not shown).
Figure 2. Effect of IN PV on OE TJ proteins: (top) western blot analysis showed that IN PV treatment (1.4%) induced a transient decrease in levels of phosphorylated occludin (65–79 kDa) in OE. From left to right, the lanes are control (0 min), 15, 30, 60 and 120 min. (bottom) Relative density ratio (mean ± SE) of phosphorylated occludin/β-actin at different time points (t = 0, 15, 30, 60 and 120 min). **Significantly different from t = 0 min at p < 0.05.
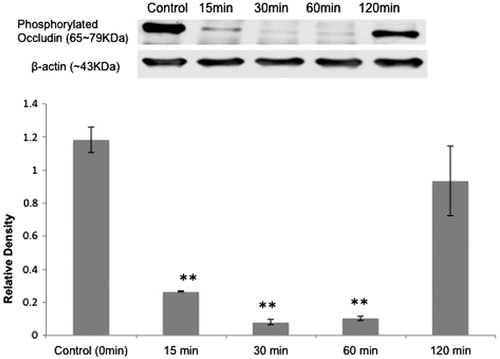
Immunofluorescence localization of occludin following PV treatment
shows occludin localization in the OE in a control rat (left), a rat 30 min after IN treatment with PV (middle), and a rat 120 min after IN PV treatment (right). In the control rat and the rat treated 120 min earlier, occludin appears as sharp crisp green bands along the edges of the apical surface of OE cells, whereas 30 min after PV treatment, staining is much less intense and discrete, with diffuse staining throughout the apical cytoplasm of OE cells, suggesting that PV treatment alters the localization and/or conformation of occludin.
Figure 3. Occludin immunofluorescence: Occludin appears as sharp crisp green bands (yellow arrows) along the edges of the apical surface of OE in control rat (left) and a rat 120 min (right) after IN PV treatment (1.4%). In the rat treated 30 min previously with IN PV (1.4%) (middle), staining is less discrete and diffuse throughout the apical cytoplasm (**) of the olfactory epithelial cells, suggesting that PV treatment reversibly alters localization or conformation of occludin. Sections were photographed at 400×.
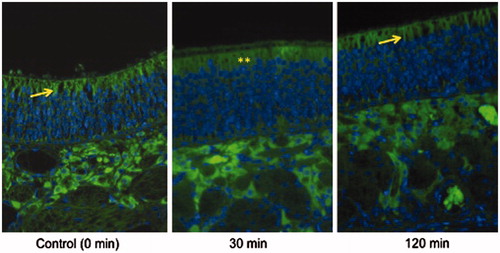
IN drug distribution
Significant fluorescence, attributable to the FD4 beads, was detected in primarily in the OE, thus suggesting that drug delivery to the CNS occurs through the OE upon IN administration (). A significant difference was identified between OE and each of the other tissues (p < 0.001).
PV enhancement of gemcitabine delivery to BECF
While 0.3% PV did not appreciably enhance gemcitabine delivery to BECF, 1.4% PV treatment significantly increased delivery of gemcitabine to BECF (). The AUC for gemcitabine in the 1.4% PV treatment group (5.54 ±0.83 μg h/mL) showed a four-fold increase, compared with 0% PV treatment (1.5 ± 0.28 μg h/mL). A similar increase was also observed for gemcitabine Cmax in BECF samples. Mean ± SD Cmax values were 0.35 ± 0.16, 0.41 ± 0.29, 0.6 ± 0.09 and 2.3 ± 1.6 µg/mL, respectively, at PV doses of 0%, 0.3%, 0.8% and 1.4%. The Tmax for 1.4% PV group was 0.5 h compared with 1 h for 0% PV group. For all groups, gemcitabine levels in BECF started returning to baseline after 2–2.5 h.
In vitro gemcitabine recovery
In vitro recovery of gemcitabine was calculated to be 12.73 ± 1.04%. Recovery was same with three different concentrations of gemcitabine.
Discussion
The current study sought to tie together several important observations. First, the potential to deliver anti-HIV nucleoside drugs to the CNS following IN administration in rodents was shown nearly two decades ago (Seki, Citation1994). One means by which nucleoside drugs are taken up is by the equilibrative nucleoside transporters, and we have previously demonstrated the presence of two equilibrative nucleoside transporters (SLC29A1 and SLC29A2 gene, formerly ENT1 and ENT2) on the surface of the olfactory mucosa (Genter et al., Citation2010). However, careful review of the literature does not suggest a strategy that might be effective in increasing the activity or expression of these transporters. Second, very recent clinical reports indicate that IN administration of a Ras inhibitor (monoterpene perillyl alcohol) was effective in extending the life span of human patients with end stage glioblastoma (da Fonseca et al., Citation2011), indicating the importance of this route of drug delivery for serious, intractable CNS disorders.
Because many of the same proteins are expressed in the BBB and in OE TJs (Wolburg & Lippoldt, Citation2002; Wolburg et al., Citation2008), and PV has previously shown to transiently increase the permeability of the BBB and BTB by altering the levels of cytoskeletal and TJ-associated proteins (Bhattacharjee et al., Citation2001, Citation2002; Platz et al., Citation2008; Wang et al., Citation2010; Xie et al., Citation2010), we hypothesized that PV would have a beneficial effect on drug delivery to CNS by reversibly increasing the permeability of olfactory epithelial TJs.
Drugs or molecules can be transported from the nose to the brain by two possible routes, transporter-mediated and paracellular transport (Frey, Citation2002; Thorne et al., Citation2004; Dhanda et al., Citation2005; Pardridge, Citation2005). Transporter-mediated drug uptake involves a balance between efflux transporters such as MRPs 1, 3, 4 and 5; Pgp, and Bcrp, as well as influx transporters such as ENT1 and 2; many of these transporters have been localized in the rat OE (Genter et al., Citation2010; Kudo et al., Citation2010; Thiebaud et al., Citation2010; Agarwal et al., Citation2013). Studies have shown gemcitabine transport is mediated by hENT1, and rat ENT1 mediates gemcitabine translocation across the BBB (Thomas et al., Citation2008; Farrell et al., Citation2009). However, active efflux limits net gemcitabine translocation, thus resulting in overall low transporter-mediated gemcitabine influx into brain (Thomas et al., Citation2008). Our goal is to enhance IN drug delivery to the brain, and since we do not know of a mechanism to upregulate or increase the expression of drug transporters in the nasal epithelia, we decided to target the paracellular route by increasing the permeability through nasal epithelial TJs.
The observation that IN-administered materials were extensively delivered to the olfactory region of the nasal cavity, coupled with the observation that peak gemcitabine concentrations in BECF were observed earlier with PV treatment (30 min) compared with the peak concentration at 60 min without PV, strongly suggests that drug delivery to the brain occurs across the OE.
In the current study, PV treatment-enhanced gemcitabine delivery to the brain in a dose-dependent manner without causing any evidence of toxicity to nasal epithelia and distant organs, even with repeated exposure. Importantly, IN-administered gemcitabine + PV resulted in gemcitabine levels in BECF that were comparable with those attained following intravenous administration of gemcitabine together with another BBB permeabilizer, RMP-7 (Apparaju et al., Citation2008). Therefore, this non-toxic and non-invasive method might prove to be useful for enhancing the delivery of other IN-administered drugs to the CNS. Other PDE inhibitors such as sildenafil (Viagra®) and vardenafil (Levitra®) have been found to increase herceptin transport and treatment efficacy in mouse metastatic brain tumor models when orally administered (Hu et al., Citation2010).
In order to understand the mechanism and time course by which PV exerts its beneficial effect on IN drug delivery, the levels of key olfactory epithelial TJ proteins were assessed using western blot analysis. We observed a rapid and transient decrease in level of the nasal epithelial TJ protein occludin (phosphorylated form) after IN PV treatment, with no change in the expression of claudin-5 over the same time course (Wang et al., Citation2010; Xie et al., Citation2010). The rapid and transient increase in paracellular permeability across nasal epithelial TJs can be explained by transient dephosphorylation of occludin leading to TJ disassembly into the cytoplasm (). It has been previously demonstrated that transient increase in in vivo nasal absorption of FITC-dextran after poly-L-arginine treatment is due to enhanced paracellular permeability via serine/threonine phosphorylation of ZO-1 and tyrosine dephosphorylation of occludin (Ohtake et al., Citation2002, Citation2003). shows the results of immunofluorescence analysis of occludin localization in the OE in a control rat (left), a rat 30 min after IN treatment with PV (middle) and a rat 120 min after IN PV treatment (right). Note that in the control rat and in the rat treated 120 min earlier, occludin appears as sharp crisp green bands along the edges of the apical surface of olfactory epithelial cells, whereas in the rat treated 30 min previously with PV, staining is much less intense and less discrete, with diffuse staining throughout the apical cytoplasm of the olfactory epithelial cells. We interpret this finding to represent transient TJ disassembly into the cytoplasm due to transient dephosphorylation or altered conformation of occludin in the OE 30 min following PV treatment, with a return to the normal pattern of expression 120 min after treatment. Both of these observations are consistent with the known biological half-life of PV, 1.5–2.2 h (Ritschel & Hammer, Citation1977). PDE10A RT-PCR analysis showed no PDE10A expression in the rat OE, thus eliminating the possibility of PDE10A inhibition in OE following IN PV treatment as a mechanism of increasing drug delivery. Our results suggest that IN PV treatment enhances paracellular permeability across nasal epithelial TJs by transient dephosphorylation or altered conformation of occludin, there by delivering significant concentration of our model drug gemcitabine to the BECF.
Conclusion
Thus, the IN route, coupled with a non-toxic, reversible epithelial TJ permeabilizer such as PV, represents a viable means to non-invasively deliver nucleoside drugs to the CNS. This strategy could dramatically improve treatment of brain tumors and CNS HIV/AIDS infections.
Declaration of interest
Funding came in part from the University of Cincinnati Center for Environmental Genetics (P30-ES006096).
Supplementary material available online Supplemental Figure 1
Supplemental Figure 1
Download PDF (52.5 KB)Acknowledgements
We gratefully acknowledge the assistance of Dr Howard Shertzer for helpful discussions; Dr M.B. Rao for statistical support; John Anneken and Courtney Huff for microdialyais study assistance; Nimita Dave and Ganesh Moorthy for help with HPLC analysis; Dr Karin Williams for assistance with immunofluorescence and Dr Susan Kasper’s lab for use of their Epson perfection V750 Pro scanner. β-Actin antibody was provided by Dr Alvaro Puga, University of Cincinnati.
References
- Agarwal S, Manchanda P, Vogelbaum MA, et al. (2013). Function of the blood-brain barrier and restriction of drug delivery to invasive glioma cells: findings in an orthotopic rat xenograft model of glioma. Drug Metab Dispos 41:33–9
- Apparaju SK, Gudelsky GA, Desai PB. (2008). Pharmacokinetics of gemcitabine in tumor and non-tumor extracellular fluid of brain: an in vivo assessment in rats employing intracerebral microdialysis. Cancer Chemother Pharmacol 61:223–9
- Argaw AT, Gurfein BT, Zhang Y, et al. (2009). VEGF-mediated disruption of endothelial CLN-5 promotes blood–brain barrier breakdown. Proc Natl Acad Sci USA 106:1977–82
- Benedict C, Hallschmid M, Hatke A, et al. (2004). Intranasal insulin improves memory in humans. Psychoneuroendocrinology 29:1326–34
- Bhattacharjee AK, Kondoh T, Ikeda M, et al. (2002). MMP-9 and EBA immunoreactivity after papaverine mediated opening of the blood brain barrier. Neuroreport 13:2217–21
- Bhattacharjee AK, Kondoh T, Nagashima T, et al. (2001). Quantitative analysis of papaverine-mediated blood-brain barrier disruption in rats. Biochem Biophys Res Commun 289:548–52
- Coskran TM, Morton D, Menniti FS, et al. (2006). Immunohistochemical localization of phosphodiesterase 10A in multiple mammalian species. J Histochem Cytochem 54:1205–13
- da Fonseca CO, Simão M, Lins IR, et al. (2011). Efficacy of monoterpene perillyl alcohol upon survival rate of patients with recurrent glioblastoma. J Cancer Res Clin Oncol 137:287–93
- Davis FG, Kupelian V, Freels S, et al. (2001). Prevalence estimates for primary brain tumors in the US by behavior and major histology groups. Neuro Oncol 3:152–8
- Davis SS, Illum L. (2003). Absorption enhancers for nasal drug delivery. Clin Pharmacokinet 42:1107–28
- De Lange SM, Van Groeningen CJ, Kroep JR, et al. (2004). Phase II trial of cisplatin and gemcitabine in patients with advanced gastric cancer. Ann Oncol 15:484–8
- Degen JW, Walbridge S, Vortmeyer AO, et al. (2003). Safety and efficacy of convection-enhanced delivery of gemcitabine or carboplatin in a malignant glioma model in rats. J Neurosurg 99:893–8
- Dhanda DS, Frey WH II, Leopold D, et al. (2005). Approaches for drug deposition in the human olfactory epithelium. Drug Del Tech 5:64–72
- Esumi Y, Mitsugi K, Seki H, et al. (1994). Placental transfer, lacteal transfer and plasma protein binding of gemcitabine. Xenobiotica 24:957–64
- Fandino J, Kaku Y, Schuknecht B, et al. (1998). Improvement of cerebral oxygenation patterns and metabolic validation of superselective intraarterial infusion of papaverine for the treatment of cerebral vasospasm. J Neurosurg 89:93–100
- Farrell JJ, Elsaleh H, Garcia M, et al. (2009). Human equilibrative nucleoside transporter 1 levels predict response to gemcitabine in patients with pancreatic cancer. Gastroenterology 136:187–95
- Frey WH II. (2002). Bypassing the blood-brain barrier to delivery therapeutic agents to the brain and spinal cord. Drug Del Tech 2:46–9
- Fujishige K, Kotera J, Omori K. (1999a). Striatum- and testis-specific phosphodiesterase PDE10A isolation and characterization of a rat PDE10A. Eur J Biochem 266:1118–27
- Fujishige K, Kotera J, Michibata H, et al. (1999b). Cloning and characterization of a novel human phosphodiesterase that hydrolyzes both cAMP and cGMP (PDE10A). J Biol Chem 274:18438–45
- Furuse M, Hirase T, Itoh M, et al. (1993). Occludin: a novel integral membrane protein localizing at tight junctions. J Cell Biol 123:1777–88
- Ge S, Song L, Pachter JS. (2005). Where is the blood–brain barrier… really? J Neurosci Res 79:421–7
- Genter MB, Burman DM, Dingeldein MW, et al. (2000). Evolution of alachlor-induced nasal neoplasms in the Long-Evans rat. Toxicol Pathol 28:770--81
- Genter MB, Krishan M, Augustine LM, et al. (2010). Drug transporter expression and localization in rat nasal respiratory and olfactory mucosa and olfactory bulb. Drug Metab Dispos 38:1644–7
- Giorgi M, Melchiorri G, Nuccetelli V, et al. (2011). PDE10A and PDE10A-dependent cAMP catabolism are dysregulated oppositely in striatum and nucleus accumbens after lesion of midbrain dopamine neurons in rat: a key step in parkinsonism physiopathology. Neurobiol Dis 43:293–303
- Griffin TW, Rasey JS, Bleyer WA. (1977). The effect of photon irradiation on blood-brain barrier permeability to methotrexate in mice. Cancer 40:1109–11
- Hawkins BT, Davis TP. (2005). The blood–brain barrier/neurovascular unit in health and disease. Pharmacol Rev 57:173–85
- Hawkins BT, Egleton RD. (2006). Fluorescence imaging of blood–brain barrier disruption. J Neurosci Methods 151:262–7
- Hu J, Ljubimova JY, Inoue S, et al. (2010). Phosphodiesterase type 5 inhibitors increase herceptin transport and treatment efficacy in mouse metastatic brain tumor models. PLoS ONE 5:e10108
- Kaku Y, Yonekawa Y, Tsukahara T, et al. (1992). Super-selective intraarterial infusion of papaverine for the treatment of cerebral vasospasm after subarachnoid hemorrhage. J. Neurosurg 77:842–7
- Kudo H, Doi Y, Fujimoto S. (2010). Expressions of the multidrug resistance-related proteins in the rat olfactory epithelium: a possible role in the phase III xenobiotic metabolizing function. Neurosci Lett 468:98–101
- Levin VA, Edwards MS, Byrd A. (1979). Quantitative observations of the acute effects of X-irradiation on brain capillary permeability: Part I. Int J Radiat Oncol Biol Phys 5:1627–31
- McMullan J, Sasson C, Pancioli A, et al. (2010). Midazolam versus diazepam for the treatment of status epilepticus in children and young adults: a meta-analysis. Acad Emerg Med 17:575–82
- Minami H, Kuwamura K, Tamaki N. (2001). Intraarterial infusion of papaverine and change of cerebral hemodynamics in symptomatic cerebral vasospasm. Kobe J Med Sci 47:169–79
- Mooradian AD, Morley JE, Kaiser FE, et al. (1989). Biweekly intracavernous administration of papaverine for erectile dysfunction. West J Med 151:515–7
- Ohtake K, Maeno T, Ueda H, et al. (2003). Poly-L-arginine enhances paracellular permeability via serine/threonine phosphorylation of ZO-1 and tyrosine dephosphorylation of occludin in rabbit nasal epithelium. Pharm Res 20:1838–45
- Ohtake K, Natsume H, Ueda H, et al. (2002). Analysis of transient and reversible effects of poly-L-arginine on the in vivo nasal absorption of FITC-dextran in rats. J Control Release 82:263–75
- Olesen SP, Crone C. (1986). Substances that rapidly augment ionic conductance of endothelium in cerebral venules. Acta Physiol Scand 127:233–41
- Pardridge WM. (2005). The blood–brain barrier and neurotherapeutics. NeuroRx 2:1–2
- Paxinos G, Watson C. (2006). The rat brain in stereotaxic coordinates. Waltham, MA: Academic Press
- Persidsky Y, Ramirez SH, Haorah J, et al. (2006). Blood-brain barrier: structural components and function under physiologic and pathologic conditions. J Neuroimmune Pharmacol 1:223–36
- Platz J, Barath K, Keller E, Valavanis A. (2008). Disruption of the blood-brain barrier by intra-arterial administration of papaverine: a technical note. Neuroradiology 50:1035–9
- Ritschel WA, Hammer GV. (1977). Pharmacokinetics of papaverine in man. Int J Clin Pharmacol Biopharm. 15:227–8
- Santoro A, Bredenfeld H, Devizzi L, et al. (2000). Gemcitabine in the treatment of refractory Hodgkin’s disease: results of a multicenter phase II study. J Clin Oncol 18:2615–9
- Sawada M, Hashimoto N, Tsukahara T, et al. (1997). Effectiveness of intra-arterially infused papaverine solutions of various concentrations for the treatment of cerebral vasospasm. Acta Neurochir (Wien) 139:706–11
- Schifitto G, Zhang J, Evans SR, et al. (2007). A multicenter trial of selegiline transdermal system for HIV-associated cognitive impairment. Neurology 69:1314–21
- Schuknecht B, Fandino J, Yuksel C, et al. (1999). Endovascular treatment of cerebral vasospasm: assessment of treatment effect by cerebral angiography and transcranial colour Doppler sonography. Neuroradiology 41:453–62
- Seki T, Sato N, Hasegawa T, et al. (1994). Nasal absorption of zidovudine and its transport to cerebrospinal fluid in rats. Biol Pharm Bull 17:1135–7
- Shipley LA, Brown TJ, Compropst JD, et al. (1992). Metabolism and disposition of gemcitabine, an oncolytic deoxycytidine analog, in mice, rats, and dogs. Drug Metab Dispos 20:849–55
- Sigmond J, Honeywell RJ, Postma TJ, et al. (2009). Gemcitabine uptake in glioblastoma multiforme: potential as a radiosensitizer. Ann Oncol 20:182–7
- Simmons RG, Phillips JB, Lojewski RA, et al. (2010). The efficacy of low-dose intranasal scopolamine for motion sickness. Aviat Space Environ Med 81:405–12
- Stamatovic SM, Keep RF, Andjelkovic AV. (2008). Brain endothelial cell–cell junctions: how to “open” the blood brain barrier. Curr Neuropharmacol 6:179–92
- Thiebaud N, Sigoillot M, Chevalier J, et al. (2010). Effects of typical inducers on olfactory xenobiotic-metabolizing enzyme, transporter, and transcription factor expression in rats. Drug Metab Dispos 38:1865–75
- Thomas FC, Rudraraju V, Taskar K, et al. (2008). Brain uptake of gemcitabine across the blood-brain barrier. AAPS J 10:T2319
- Thorne RG, Pronk GJ, Padmanabhan V, Frey WH II. (2004). Delivery of insulin-like growth factor-I to the rat brain and spinal cord along olfactory and trigeminal pathways following intranasal administration. Neuroscience 127:481–96
- Ueno M. (2007). Molecular anatomy of the brain endothelial barrier: an overview of the distributional features. Curr Med Chem 14:1199–206
- Wang ZH, Xue YX, Liu YH. (2010). The modulation of protein kinase A and heat shock protein 70 is involved in reversible increase of blood-brain tumor permeability induced by papaverine. Brain Res Bull 20:367–73
- Wolburg H, Lippoldt A. (2002). Tight junctions of the blood–brain barrier: development, composition and regulation. Vascul Pharmacol 38:323–37
- Wolburg H, Wolburg-Buchholz K, Sam H, et al. (2008). Epithelial and endothelial barriers in the olfactory region of the nasal cavity of the rat. Histochem Cell Biol 130:127–40
- Wong MH, Robertson K, Nakasujja N, et al. (2007). Frequency of and risk factors for HIV dementia in an HIV clinic in sub-Saharan Africa. Neurology 68:350–5
- Xie H, Xue Y, Liu L, et al. (2010). Endothelial-monocyte-activating polypeptide II increases blood–tumor barrier permeability by down-regulating the expression levels of tight junction associated proteins. Brain Res 1319:13–20
- Yamamoto BK, Pehek EA. (1990). A neurochemical heterogeneity of the rat striatum as measured by in vivo electrochemistry and microdialysis. Brain Res 506:236–42
- Yoshimura S, Hashimoto N, Goto Y, et al. (1996). Intraarterial infusion of high-concentration papaverine damages cerebral arteries in rats. Am J Neuroradiol 17:1891–4
- Young JT. (1981). Histopathologic examination of the rat nasal cavity. Fundam Appl Toxicol 1:309–12