Abstract
To further develop cholesterol-modified pullulan self-aggregated nanoparticles (CHSPNs) as a drug nanocarrier, CHSP was synthesized and characterized. Its cholesterol degree determined by 1H NMR was 5.2 cholesterol groups per hundred glucose units. CHSPNs were prepared in aqueous media and characterized by dynamic laser light-scattering (DLS), zeta potential and transmission electron microscopy (TEM). These nanoparticles were almost spherical in shape, and the zeta potentials of CHSPNs were near zero in aqueous media. CHSPNs can be stable at least 2 months with no significant size and zeta potential changes. Single dose toxicity test in mice was investigated for the safety evaluation of CHSPNs as a drug nanocarrier, and the result showed CHSPNs were well tolerated at the dose of 200 mg/kg in mice. Epirubicin (EPI)-loaded CHSPNs (EPI-CHSPNs) were prepared and the in vivo pharmacokinetics and biodistribution were studied. Compared with the EPI solution, EPI-CHSPNs have exhibited higher plasma drug concentration, longer half-life time (t1/2) and the larger area under-the-curve (AUC). Moreover, the drug level of EPI-CHSPNs increased in liver and decreased in heart. The results indicated that CHSPNs were stable, safe and may be a promising drug delivery carrier.
Introduction
Nanomaterials are structures with at least one dimension of 100 nm or less. These materials have specific physic-chemical properties different to bulk materials of the same composition, and are increasingly being used for commercial purposes such as fillers, catalysts, semiconductors, cosmetics, microelectronics and drug carriers (Landsiedel et al., Citation2010). Using the nanoparticles (NPs) to deliver drugs, the absorption of poorly water-soluble pharmaceuticals may be improved and led to prolong pharmacological effect, enhance drug bioavailability and increase the patient’s compliance (Gonçalves et al., Citation2010). The unusual physic-chemical properties of NPs are attributable to their small size, chemical composition, surface structure, solubility, shape and aggregation. The biological impacts and the biokinetics of NPs are dependent on their physicochemical parameters, which can modify cellular uptake, protein binding, clearance, metabolism, translocation from portal of entry to the target site. However, the properties may also cause new side effects and differential toxicity profiles (Nel et al., Citation2006; Vega-Villa et al., Citation2008). The pharmacokinetic profiles of the parent drug and the drug encapsulated in the NPs are often different. In vivo systems are extremely complicated and the data of nanotoxicity from the cell culture systems are imperfect. Therefore, it is very important to understand the relationship between the NP’s physic-chemical properties and its behavior in vivo (Fischer & Chan, Citation2007; Herkenne et al., Citation2008).
Pullulan, a very important neutral and linear natural polysaccharide, has many advantages as a macromolecular drug carrier, e.g. highly water-soluble, non-toxic, non-mutagenic, edible, lacks immunogenicity, and usefulness as a plasma expander, multiple hydroxyl groups that can readily be modified chemically (Leathers, Citation2003; Liu et al., Citation2008; Singh et al., Citation2008). Many investigations have reported that some amphiphilic pullulan derivatives can form self-aggregated NPs to be used as the carrier for drug delivery (Zhang et al., Citation2009; Yang et al., Citation2010; Lee et al., Citation2012; Yang et al., Citation2014). Cholesterol-modified pullulan (CHP), as one of amphiphilic pullulan derivatives, can be synthesized using 1,6-hexyldiisocyanate linkages by the reaction between cholesterol and pullulan (Akiyoshi et al., Citation1993), and its self-aggregated CHP NPs can be used as drug delivery systems (Akiyoshi et al., Citation1998; Ikuta et al., Citation2002; Sunamoto et al., Citation2006; Shimoda et al., Citation2012) or artificial molecular chaperones (Nomura et al., Citation2003; Asayama et al., Citation2008). The outstanding characteristics of cholesterol-modified pullulan attract our group to engage in this research. In this article, we synthesized CHSP using succinic acid linker according to our previous report (Yang et al., Citation2010). The exogenous 1,6-hexyl diisocyanate was replaced by succinic acid, a small endogenous molecule of the body’s metabolic process, which provides a new strategy for the effective and safe synthesis CHSP. The reports about CHSPNs are gradually increasing (Yang et al., Citation2010; Tao et al., Citation2012a, Citationb; Yang, et al., Citation2014), but the relationship between the physic-chemical characteristics of the CHSPNs and their in vivo behavior is seldom reported. Herein, CHSP was synthesized and characterized by FT-IR, 1H NMR and differential scanning calorimetry (DSC), and then CHSPNs with moderate size and low potential were prepared by probe sonication in distilled water. The storage stability of CHSPNs was studied in the aqueous medium, and the acute toxicity of CHSPNs was evaluated in mice. Moreover, compared to the epirubicin solution, the pharmacokinetics and biodistribution of EPI-CHSPNs were also assessed in rats. By the above-mentioned work, our groups hope to further promote the application of CHSP materials in medical field.
Methods
Materials
Pullulan (Mw 200000) was purchased from Hayashibara Tokyo (Japan). 4-Dimethylaminopyridine (DMAP) and 1-ethyl-3-[3-(dimethylamino) propyl] carbodiimide (EDC) were purchased from Sigma Co. (St. Louis, MO). Epirubicin·HCl (EPI·HCl) was purchased from Hisun Pharmaceutical Co. (Zhejiang, China). All reagents were of analytical grade and used without further purification. ICR mice and Wistar rats were purchased from experimental animal center of Hebei Province (Shijiazhuang, China). All animal experiments were performed in compliance with the requirements of the National Act of the People’s Republic of China on the use of experimental animals.
Synthesis and characteristic of CHSP
CHSP was synthesized according to the method described in literatures (Yang et al., Citation2010). Briefly, the pullulan powder (0.5 g) was dissolved in 30 mL dry DMSO. The cholesterol succinate (CHS) in dried DMSO was activated by the addition of EDC (1.5 equiv CHS) and DMAP (1 equiv CHS). The amounts of activated CHS (0.10 mol/mol sugar residues of pullulan) were added into the dry DMSO solution containing pullulan. The mixture was reacted by stirring at 45 °C for 48 h. Then, the reactant mixture was dropped into ethanol and the precipitate was collected by filtration and washed with ethanol, tetrahydrofuran and diethyl ether, respectively. Then, the sample was dried under vacuum to obtain CHSP conjugates. The chemical structure of CHSP was confirmed using FT IR (Thermo, Nicolet, Los Angeles, CA) and 1H NMR (VARINA INOVA 500 MHz, USA). The degree of substitute (DS) of cholesterol residues per 100 glucose units in pullulan could be calculated by 1H NMR using DMSO-d6 as the solvent. The degree of substitute (DS) of cholesterol residues per 100 glucose units in pullulan could be calculated by the ratio of methylene groups protons (2.53 ppm) to sugar protons (C1 position of α-1,6 and α-1,4 glycosidic bonds, 4.68 and 5.00 ppm) as the following Equation (1):
The physical status of CHSP was investigated by DSC 2910 MDSC V4.4 E (USA). For this, the small amount (2–7 mg) of sample was sealed in the aluminum pan and the temperature was raised at 10 °C/min from 50 to 250 °C under nitrogen (20 mL/min) atmosphere.
Preparation and characterization of CHSPNs and EPI-CHSPNs
CHSP conjugates were suspended and swollen in distilled water under gentle shaking at 37 °C for 24 h to give a milky suspension, followed by sonication using a probe type sonifier (SCIENTZ LCD JY 92, Ningbo, China) at 100 W for 2 min. The sonication step was repeated 3 times until the desired size value had been reached. To prevent the sample solution from heat built-up during the sonication, the ice water bath and pulse function were used (pulse on 2.0 s, pulse off 2.0 s). The solution of self-aggregated NPs was passed through membrane filter (pore size: 0.45 μm [Beijing Jingke Hongda Biotechnology Co., Ltd. China], Millipore dialysis tube [Viskase, USA]) and stored at 4 °C. Subsequently, CHSPNs were dispersed in distilled water and 10% fetal bovine serum (FBS), respectively, with CHSP concentration of 1 mg/mL to carry out the later experiments.
EPI-loaded CHSP (DS 5.2) self-aggregated NPs were prepared by a dialysis method (Jeong et al., Citation2006; Wang et al., Citation2008). Briefly, EPI·HCl and CHSP were dissolved in DMSO while the amount of triethylamine was added under stirring, and then the DMSO and free EPI were removed by dialyzing (Millipore dialysis tube, molecular weight cutoff 12–14 kDa, USA). The dialyzed liquids were exchanged each 1 h for the first 3 h and then each 2 h for another 6 h to against 1 L deionized water, followed by sonication using a probe type sonifier at 100 W for 2 min to obtain EPI-loaded CHSP self-aggregated NPs. The dust and impurity in the sample solution were removed by passing through a filter (0.45 μm, Millipore). The EPI-loaded CHSPNs were obtained after lyophillization through the LGJ-10 freezer-drier (Beijing Huaxing Technology Development Co., Ltd., Songyuan, China). The drug loading contents (LC) was determined by HPLC analysis, the LC values can be calculated as following Equation (2), and the LC of EPI-CHSPNs was 7.8% (w/w) which was used in vivo experiment.
The particle size and zeta potential of CHSPNs or EPI-loaded CHSPNs were studied by dynamic light scattering (Malvern Instruments Ltd., Zeta sizer 2000, Worcestershire, UK). The morphology of NPs was observed using TEM (JEM-100 C, Japan).
The stability of CHSPNs in water
In order to study the stability of CHSPNs, the above CHSPNs solution was stored for 2 months at 4 °C. Macroscopic characteristics of NPs dispersions such as opalescence and precipitation were observed. The size and zeta potential of CHSPNs were also determined by dynamic light scattering method once a month, and all measurements were performed in triplicates.
In vivo toxicity
The toxicity test of CHSPNs was carried out in vivo to clarify its applicability in a biosystem according to the previous reported methods (Yoksan & Chirachanchai, Citation2008; Sonaje et al., Citation2009). Adult male (22–24 g) and female ICR mice (18–22 g) were randomly divided into two groups with each of 10 mice. The experimental group was received single intravenous (i.v.) dose of blank CHSPNs (200 mg/kg); the control group was treated with single i.v. dose of normal saline. All animals were fed with normal diet and water. Animals were observed carefully for the onset of any signs of toxicity and monitored for changes in food intake and body weight at 1, 8 and 15 d. After sacrificed at 15 d, internal organs of each animal were cut and observed grossly for the evaluation toxicity of CHSPNs. Specimens of major organs such as heart, liver, spleen, lung and kidney were fixed in 10% phosphate buffered formalin, embedded in paraffin, sectioned and stained with hematoxylin and eosin (H&E).
In vivo pharmacokinetics
The in vivo pharmacokinetics was measured with female Wistar rats of 180–220 g and 4–6 weeks old, which were held in air-conditioned facility and provided with standard food and filtered water. Animals were randomly assigned to two groups with each of six rats, which received an intravenous injection via the tail vein of free EPI·HCl and the EPI-CHSPNs solution in saline at 10 mg/kg equivalent dose, respectively. The EPI content of the loaded CHSPNs was about 7.8% (w/w). At selected times (t = 0.0167, 0.0833, 0.167, 0.5, 1, 2, 4, 8, 12, 24 and 48 h), the blood samples were collected with heparinized tube and centrifuged at 3000 rpm for 15 min to separate the plasma. The plasma samples were stored immediately at −20 °C until analysis.
The plasma (200 µL) was mixed with dichloromethane–methanol (4:1, v/v) on a vortex-mixer for 3 min to extract the drug. Upon centrifugation at 10 000 rpm for 15 min, the upper aqueous layer was removed and the organic layer was transferred to a tube and evaporated under nitrogen at 50 °C. The residue was dissolved in 100 µL anhydrous methanol by vortex. A Hypersil BDS C18 column (4.6 mm × 250 mm, 5 μm) was used for the HPLC analysis and the mobile phase (0.02 M KH2PO4/CH3CN/CH3OH = 49:17:34 v/v/v) was delivered at a rate of 1 mL/min. Sample (20 μL) was injected and the column effluent was monitored with a UV detector at 232 nm. The main pharmacokinetic parameters were calculated.
Tissue biodistribution study
To assess the effect of EPI-CHSPNs on tissue distribution of EPI, female Wistar rats, 180–220 g and 4–6 weeks old, were randomly assigned to two groups with 15 rats in each. Each group received an intravenous injection of free EPI and EPI-CHSPNs via the tail vein at an equivalent dose of 10 mg/kg, respectively. Each group consisted of three sets, each having five rats. At 6 h, 24 h and 48 h after drug injection, animals in each set were killed and various tissues such as heart, liver, spleen, lung and kidney were collected. Tissue samples were washed in saline, blotted with paper towel to remove excess fluid, weighed and homogenized with PBS, pH 7.4. The mixture was extracted and evaporated in the same way as the blood samples. Then, the dried residue was dissolved in 200 μL of methanol and quantified fluorometrically at λex 470 nm and λem 589 nm (SANCO, 970-CRT, China) (Li et al., Citation2010). To correct for background fluorescence, a standard curve was obtained by spiking tissue extracts derived from rats that had not received EPI.
Statistical analysis
All data were shown as a mean ± SD. Statistical analysis was conducted using the Students’t-test and p values less than 0.05 was considered as statistically significant.
Results and discussion
Characterization of CHSP
As shown in , it is easy to synthesize hydrophobic pullulan derivative because of its three free hydroxyl groups on each glucose unit. By means of replacing the hydroxyl groups of the glucose unit with CHS groups, CHSP was prepared and characterized by IR spectroscopy to identify carbonyl groups. FTIR spectrum of CHSP () shows a new stretching vibration band relative to C=O bond at about 1735 cm−1 and the enhanced stretching vibration band relative to –CH3, –CH2– groups at about 2941 cm−1. The observation was consistent with the existence of ester bond and methylene between pullulan and cholesterol residues, which indicated that CHSP was synthesized successfully, and its similar to literature (Yang et al., Citation2010). As shown in , the 1H NMR spectrum of CHSP permitted the identification of the protons corresponding to the pullulan chain at: 0.59–1.20 ppm (the H signal of cholesterol) (Zipser et al., Citation1998; Kim et al., Citation2012), the singlet at 0.63 and 0.96 ppm which is assigned to the two angular methyl groups (18-CH3 and 19-CH3), the peak at 0.83 ppm (26, 27-CH3), the peak at 0.88 ppm (21-CH3), 2.48 (DMSO-d6) and 2.53 (2 methylene groups, –OCCH2CH2CO–) ppm. The peak at 2.53 ppm is the proton signal of two methylene groups, this peak plays a key role in supporting the conclusion that pullulan was successfully reacted with cholesterol by succinic acid linker. Moreover, the degree of substitute (DS) of cholesterol residues per 100 glucose units in pullulan could be calculated by the ratio of methylene groups protons (2.53 ppm) to sugar protons (C1 position of α-1,6 and α-1,4 glycosidic bonds, 4.68 and 5.00 ppm (Sivakumar & Panduranga Rao, Citation2003; Yang et al., Citation2010)). The results of DS of cholesterol moiety were 5.2, which was used to prepare CHSPNs and EPI-CHSPNs in this study.
DSC studies were performed to further investigate the synthetic CHSP, so as to distinguish between physical mixture (pullulan and succinate cholesterol) and CHSP, ensure the successful synthetic CHSP. shows the DSC thermograms of pullulan, succinate cholesterol (CHS), CHSP and physical mixture. The melting endothermic peak of succinate cholesterol appears at 175 °C. No obvious melting peak is detected in CHSP sample but the melting peak appears at 175 °C in the physical mixture sample. Moreover, pullulan shows a broad endotherm at around 90 °C whereas CHSP changes at around 70 °C. All above-mentioned facts conclude that CHSP is synthesized successfully.
Particle size, zeta potential and TEM investigations of NPs
The morphology of NPs stained by phosphotungstic acid in the transmission electron microscope photographs is shown in . As can be seen from the figure, CHSPNs and EPI-CHSPNs were nearly spherical in shape and uniform sized. It was known that the particle size distribution was one of the most important characteristics for the evaluation of colloidal systems. The size and the size distributions of CHSPNs and EPI-CHSPNs in the aqueous media were measured by DLS. As shown in , the mean diameters of CHSPNs and EPI-CHSPNs were 51.8 ± 4.6 nm and 160.6 ± 37.7 nm with the narrow size distributions (PDI < 0.3). The size of the NPs increased when the EPI was loaded with CHSPNs.
Table 1. Size, PDI and zeta potential of CHSPNs and EPI-CHSPNs ( ± s, n = 5).
The zeta potential of NPs was a useful tool to predict the physical storage stability of colloidal systems. As shown in , the zeta potentials of CHSPNs and EPI-CHSPNs in distilled water were −1.253 ± 1.161 and −1.326 ± 1.211 mV. To mimic the circulation in vivo, we observed the zeta potentials of NPs in 10% FBS, which had a composition very similar to the body liquids. The zeta potentials of CHSPNs and EPI-CHSPNs in 10% FBS were −1.382 ± 0.597 and −1.522 ± 0.892 mV, respectively. Levchenko et al. demonstrated that the blood clearance rate of NPs with different surface charge was significantly different (Levchenko et al., Citation2002). Neutral NPs would exhibit a decreased rate of macrophage phagocytosis system (MPS) uptake. However, MPS is the major contributor for the clearance of NPs, thus the reducing rate of MPS uptake could be considered as the best strategy for prolonging the circulation of NPs (Li & Huang, Citation2008). The zeta potentials of CHSPNs and EPI-CHSPNs were near zero, therefore, EPI-CHSPNs would have a long-circulating action.
The storage stability of CHSPNs
NPs stability is an important facet of preparation and a necessary step in the development process, which will indicate the potentiality of industry production. The size, charge, chemical composition, surface characteristics affect the stability of NPs (Pavlin & Bregar, Citation2012). Those factors also affect drug-loading efficiency, the drug release profile, the pharmacokinetics and toxicity of NPs (Moghimi et al., Citation2012). In our study, CHSPNs dispersions maintained slight opalescence within 2 months. As shown in , the size, size distribution and zeta potential of CHSPNs showed no significant changes during 2 months. Therefore, it could be concluded that CHSPNs in aqueous media were stable for at least 2 months at 4 °C. The literature reported that stabilization of colloidal systems is traditionally viewed as arising from either electrostatic or steric effects (Lourenco et al., Citation1996; Simakov & Tsur, Citation2007). The results showed that a steric stabilization may occur and play a key role in improving the stability of the CHSPNs.
In vivo toxicity of CHSPNs
To evaluate whether i.v. administration (at dose of 200 mg/kg) of CHSPNs was associated with any toxicity in vivo, animals were treated with a single dose of empty CHSPNs. shows the experimental data of the CHSPNs and control group. The body weight and food intake data observed for 1, 8, and 15 d, including the rat survival, are to evaluate CHSPNs acute toxicity. It can be seen from that there were no significant differences between two groups whether in body weight or food intake. The clinical signs, such as diarrhea, fever and other systemic symptoms in the CHSPNs group, were not found during the whole experiment process and the mortality rate was zero. Moreover, the necropsy showed no obvious inflammatory reactions and pathological changes. Pathological changes in the major organs including heart, liver, spleen, lung, kidney, stomach and intestinal segments were scarcely observed at 15 d. The microscopic examination of major organs including heart, liver, spleen, lung and kidney sections stained with H&E and are presented in . All the above-mentioned results indicated that no apparent toxicity of the CHSPNs was found in the experimental animals after i.v. at dose of 200 mg/kg.
In vivo pharmacokinetics
shows the blood concentration–time profiles of epirubicin after a single i.v. injection of EPI · HCl or EPI-CHSPNs (10 mg/kg EPI equiv.) in female rats. The non-compartmental pharmacokinetic parameters are presented in . The peak concentration of total EPI in plasma is 9.71 ± 1.07 mg/L at 1 min after injection and then decreased to nearly undetectable levels after 24 h. The free EPI rapidly disappears from the circulation due to its short half-life (t1/2). In this study, the t1/2 of free EPI is 6.95 ± 0.51 h, which is consistent with that of 7.3 ± 1.8 h of doxorubicin (Gustafson et al., Citation2002). Whereas the t1/2 of EPI-CHSPNs is 2.8-fold longer than that of free EPI. The clearance of EPI-CHSPNs is 0.23 ± 0.09 L/h, which is 3.5-fold smaller than free EPI. Moreover, the mean residence time (MRT) of the drug in the plasma is also increased 2.8 times. Therefore, EPI-CHSPNs appear the longer t1/2 and MRT than that of EPI solution in rats. The longer circulation time of EPI-CHSPNs in plasma may be due to its low zeta potential and moderate size (Li & Huang, Citation2008). At the same time, the EPI-CHSPNs gave significantly higher area under-the-curve (AUC). As shown in , the AUC of EPI and EPI-CHSPNs are 19.38 ± 8.08 and 48.36 ± 15.76 mg· h/L, respectively. Compared to free EPI solution, the AUC of EPI-CHSPNs increased 2.5-fold. The slow release of EPI from CHSPNs in the blood suggested that the bioavailability of EPI improved when it is loaded into CHSPNs.
Figure 6. Plasma drug concentration of EPI and EPI/CHSPNs after i.v. injection in rats at a single equivalent dose of 10 mg/kg.
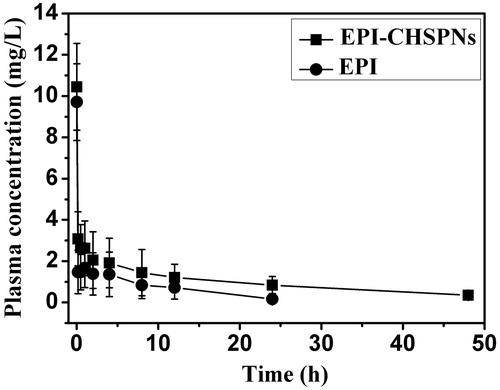
Table 2. Pharmacokinetic parameters of EPI and EPI-CHSPNs i.v. injected in rats at the equivalent 10 mg/kg dose.
Tissue biodistribution
The biodistribution results of free EPI and EPI-CHSPNs (10 mg/kg EPI equiv.) in solid tissues such as heart, liver, spleen, lung and kidney are shown in . Free EPI was mainly distributed to the heart and kidney after injection, which might cause acute toxicity and would be rapidly eliminated from the circulation. Compared with free EPI, the amount of drug in the heart and kidney for EPI-CHSPNs dropped by 3.3- and 1.7-fold at 6 h, respectively. Moreover, the amount of drug in liver increased by 1.5 and 2.4 times at 6 and 24 h, respectively, which indicated that CHSPNs show a high targeting efficiency and a long retention time in liver. The lower drug amount for EPI-CHSPNs in heart than the control in tissue biodistribution will be advantageous to reduce the toxicity of EPI.
Conclusions
In this article, cholesterol was grafted onto pullulan and amphiphilic pullulan derivative was synthesized and characterized. CHSP self-assembled NPs were prepared, which were stable with moderate size and low potential in aqueous media for 2 months. The acute toxicity investigation in vivo proved that CHSPNs were safe in mice at 200 mg/kg. Moreover, the pharmacokinetics and tissue distribution of EPI entrapped in CHSPNs after intravenous injection into rats changed compared to free EPI. The uptake of EPI significantly increased in plasma and liver, but decreased in heart. It is concluded that CHSPNs can modify the biodistribution of EPI, which is advantageous to enhance the therapeutic index and reduce the toxicity of EPI.
Declaration of interest
This work was supported by the Natural Science Foundation of Hebei Province (No. H2012201069; B2013201181), the Science and Technology Planning Project of Hebei Province (No. 13272705) and the Natural Science Foundation of Hebei University, China. (No. 2010-193).
References
- Akiyoshi K, Deguchi S, Moriguchi N, et al. (1993). Self-aggregates of hydrophobized polysaccharides in water. Formation and characteristics of nanoparticles. Macromolecules 26:3062–8
- Akiyoshi K, Kobayashi S, Shichibe S, et al. (1998). Self-assembled hydrogel nanoparticle of cholesterol-bearing pullulan as a carrier of protein drugs: Complexation and stabilization of insulin. J Control Rel 54:313–20
- Asayama W, Sawada S, Taguchi H, Akiyoshi K. (2008). Comparison of refolding activities between nanogel artificial chaperone and GroEL systems. Int J Biol Macromol 42:241–6
- Fischer HC, Chan WCW. (2007). Nanotoxicity: the growing need for in vivo study. Curr Opin Biotechnol 18:565–71
- Gonçalves C, Torrado E, Martins T, et al. (2010). Dextrin nanoparticles: studies on the interaction with murine macrophages and blood clearance. Coll Surf B: Biointerf 75:483–9
- Gustafson DL, Rastatter JC, Colombo T, Long ME. (2002). Doxorubicin pharmacokinetics: macromolecule binding, metabolism, and excretion in the context of a physiologic model. J Pharmaceut Sci 91:1488–501
- Herkenne C, Alberti I, Naik A, et al. (2008). In vivo methods for the assessment of topical drug bioavailability. Pharmaceut Res 25:87–103
- Ikuta Y, Katayama N, Wang L, et al. (2002). Presentation of a major histocompatibility complex class 1–binding peptide by monocyte-derived dendritic cells incorporating hydrophobized polysaccharide–truncated HER2 protein complex: implications for a polyvalent immuno-cell therapy. Blood 99:3717–24
- Jeong YI, Kim SH, Jung TY, et al. (2006). Polyion complex micelles composed of all-trans retinoic acid and poly (ethylene glycol)-grafted-chitosan. J Pharmaceut Sci 95:2348–60
- Kim JH, Li Y, Kim MS, et al. (2012). Synthesis and evaluation of biotin-conjugated pH-responsive polymeric micelles as drug carriers. Int J Pharmaceut 427:435–42
- Landsiedel R, Ma-Hock L, Kroll A, et al. (2010). Testing metal-oxide nanomaterials for human safety. Adv Mater 22:2601–27
- Leathers TD. (2003). Biotechnological production and applications of pullulan. Appl Microbiol Biotechnol 62:468–73
- Lee SJ, Hong GY, Jeong YI, et al. (2012). Paclitaxel-incorporated nanoparticles of hydrophobized polysaccharide and their antitumor activity. Int J Pharmaceut 433:121–8
- Levchenko TS, Rammohan R, Lukyanov AN, et al. (2002). Liposome clearance in mice: the effect of a separate and combined presence of surface charge and polymer coating. Int J Pharmaceut 240:95–102
- Li L, Gao FP, Tang HB, et al. (2010). Self-assembled nanoparticles of cholesterol-conjugated carboxymethyl curdlan as a novel carrier of epirubicin. Nanotechnology 21:265601 (11pp)
- Li SD, Huang L. (2008). Pharmacokinetics and biodistribution of nanoparticles. Mol Pharmaceut 5:496–504
- Liu Z, Jiao Y, Wang Y, et al. (2008). Polysaccharides-based nanoparticles as drug delivery systems. Adv Drug Deliv Rev 60:1650–62
- Lourenco C, Teixeira M, Simões S, Gaspar R. (1996). Steric stabilization of nanoparticles: size and surface properties. Int J Pharmaceut 138:1–12
- Moghimi SM, Hunter A, Andresen T. (2012). Factors controlling nanoparticle pharmacokinetics: an integrated analysis and perspective. Ann Rev Pharmacol Toxicol 52:481–503
- Nel A, Xia T, Mädler L, Li N. (2006). Toxic potential of materials at the nanolevel. Science 311:622–7
- Nomura Y, Ikeda M, Yamaguchi N, et al. (2003). Protein refolding assisted by self-assembled nanogels as novel artificial molecular chaperone. FEBS Lett 553:271–6
- Pavlin M, Bregar VB. (2012). Stability of nanoparticle suspensions in different biologically relevant media. Dig J Nanomater Bios 7:1389–400
- Shimoda A, Yamamoto Y, Sawada SI, Akiyoshi K. (2012). Biodegradable nanogel-integrated hydrogels for sustained protein delivery. Macromol Res 20:266–70
- Simakov S, Tsur Y. (2007). Surface Stabilization of nano-sized titanium dioxide: improving the colloidal stability and the sintering morphology. J Nanoparticle Res 9:403–17
- Singh RS, Saini GK, Kennedy JF. (2008). Pullulan: Microbial sources, production and applications. Carbohydr Polym 73:515–31
- Sivakumar PA, Panduranga Rao K. (2003). The use of cholesteryl pullulan for the preparation of stable vincristine liposomes. Carbohydr Polym 51:327–32
- Sonaje K, Lin YH, Juang JH, et al. (2009). In vivo evaluation of safety and efficacy of self-assembled nanoparticles for oral insulin delivery. Biomaterials 30:2329–39
- Sunamoto J, Ushio K, Lai DT. (2006). Folate-modified cholesterol-bearing pullulan, a new cancer-targeted nanoparticle drug carrier: synthesis and applications. J Bioactive Compatible Polym 21:603–17
- Tao X, Zhang Q, Ling K, et al. (2012a). Effect of pullulan nanoparticle surface charges on HSA complexation and drug release behavior of HSA-bound nanoparticles. PloS One 7:e49304 (13pp)
- Tao X, Zhang Q, Yang W, Zhang Q. (2012b). The interaction between human serum albumin and cholesterol-modified pullulan nanoparticle. Curr Nanosci 8:830–7
- Vega-Villa KR, Takemoto JK, Yáñez JA, et al. (2008). Clinical toxicities of nanocarrier systems. Adv Drug Deliv Rev 60:929–38
- Wang YS, Jiang Q, Li RR, et al. (2008). Self-assembled nanoparticles of cholesterol-modified O-carboxymethyl chitosan as a novel carrier for paclitaxel. Nanotechnology 19:145101 (8pp)
- Yang WZ, Chen HL, Gao FP, et al. (2010). Self-aggregated nanoparticles of cholesterol-modified pullulan conjugate as a novel carrier of mitoxantronep. Curr Nanosci 6:298–306
- Yang W, Wang M, Ma L, et al. (2014). Synthesis and characterization of biotin modified cholesteryl pullulan as a novel anticancer drug carrier. Carbohydr Polym 99:720–7
- Yoksan R, Chirachanchai S. (2008). Amphiphilic chitosan nanosphere: studies on formation, toxicity, and guest molecule incorporation. Bioorganic Med Chem 16:2687–96
- Zhang HZ, Gao FP, Liu LR, et al. (2009). Pullulan acetate nanoparticles prepared by solvent diffusion method for epirubicin chemotherapy. Coll Surf B: Biointerf 71:19–26
- Zipser B, Bradford JJ, Hollingsworth RI. (1998). Cholesterol and its derivatives, are the principal steroids isolated from the leech species Hirudo medicinalis. Comparat Biochem Physiol Part C: Pharmacol, Toxicol Endocrinol 120:269–82