Abstract
Context: Metformin hydrochloride is a biguanide derivative widely used for the treatment of type 2 diabetes, prescribed nearly to 120 million people worldwide. Metformin has a relatively low oral bioavailability (about 50–60%). Although the major effect of metformin is to decrease hepatic glucose output as an antihyperglycemic agent, its inhibitory effects on the proliferation of some cancer cells (e.g. prostate, breast, glioma cells) have been demonstrated in the cell culture studies. Development of novel formulation (e.g. microparticles, nanoparticles) strategies for metformin might be useful to improve its bioavailability, to reduce the dosing frequency, to decrease gastrointestinal side effects and toxicity and to be helpful for effective use of metformin in cancer treatment.
Objective: The main aim of this review is to summarize metformin HCl-loaded micro- and nanoparticulate drug delivery systems.
Method: The literature was rewieved with regard to the physicochemical, pharmacological properties of metformin, and also its mechanism of action in type 2 diabetes and cancer. In addition, micro- and nanoparticulate drug delivery systems developed for metformin were gathered from the literature and the results were discussed.
Conclusion: Metformin is an oral antihyperglycemic agent and also has potential antitumorigenic effects. The repeated applications of high doses of metformin (as immediate release formulations) are needed for an effective treatment due to its low oral bioavailability and short biological half-life. Drug delivery systems are very useful systems to overcome the difficulties associated with conventional dosage forms of metformin and also for its effective use in cancer treatment.
Introduction
Metformin hydrochloride is a biguanide derivative widely used for the treatment of type 2 diabetes, prescribed nearly to 120 million people worldwide (Sweetman, Citation2007; Viollet et al., Citation2012). Biguanides are the active compound of the French Lilac (Galega officinalis). They were first identified in the 1920s and used as therapeutic agents in the 1950s. Metformin was approved as an antihyperglycemic agent in Britain in 1958, Canada in 1972 and the United States in 1995 (Dowling et al., Citation2011). Metformin is recommended by European Association for the Study of Diabetes, and the American Diabetes Association as the firstline therapy for type 2 diabetes (Viollet et al., Citation2012).
The major effect of metformin is to decrease hepatic glucose output as an antihyperglycemic agent. Furthemore, it increases insulin-mediated glucose utilization in peripheral tissues (e.g. liver and muscle), decreases the glucose absorption in the small intestine and lowers the plasma free fatty acid concentrations, thereby reducing substrate availability for gluconeogenesis (Grzybowska et al., Citation2011; Viollet et al., Citation2012; McCulloch, Citation2015). As a result, it decreases the blood glucose concentrations in type 2 diabetes and do not induce overt hypoglycemia. Recently, metformin has found to be effective in the treatment of various cancers especially prostate, colon and breast cancer (Dowling et al., Citation2011).
Following oral administration, metformin is mainly absorbed from the upper small intestine and has a relatively low bioavailability. The absolute bioavailability of metformin is about 50 to 60%. Its incomplete absorption is improved by using convenient drug delivery systems, such as bioadhesive and gastroretentive drug delivery systems (Adikwu et al., Citation2003; Sweetman, Citation2007; Boldhane & Kuchekar, Citation2009; Murphy et al., Citation2012; Raparla & Talasila, Citation2012).
Its biological half-life (t1/2) is in the range of 0.9–2.6 h. Therefore, repeated applications of high doses of metformin (500 mg two or three times daily, or 850 mg once or twice daily with or after meals) are needed for an effective treatment. As a result, patient compliance is reduced and/or the incidence of side effects, such as diarrhoea, nausea, anorexia, vomiting, weight loss, and taste disturbance, is increased (Sweetman, Citation2007; Corti et al., Citation2008). Moreover, lactic acidosis, which is sometimes fatal, has occurred with biguanides (Sweetman, Citation2007). Development of novel formulation strategies for metformin might be useful to improve its bioavailability, to reduce the dosing frequency and to decrease gastrointestinal side effects and toxicity (Marathe et al., Citation2000; Cetin et al., Citation2013; Basavaraj & Betageri, Citation2014). Also, these systems may be helpful for effective use of metformin in cancer treatment (Alexis et al., Citation2010).
Particulate drug delivery systems (e.g. polymeric microparticles, polymeric nanoparticles) have several advantages over the conventional dosage forms. These include higher local drug concentrations, less variation in the gastrointestinal transit times, low variability among individuals, low risk of dose dumping, reduced side effects, possibility of different routes of administration (e.g. oral, inhalation, parenteral), and also hydrophilic and hydrophobic drug loading (Amorim & Ferreira, Citation2001; Gelperina et al., Citation2005; Gundogdu & Cetin, Citation2014). Of the particulate dosage forms, especially nanoparticulate drug delivery systems are unique, and are used as a promising approach for the treatment of cancer over the last two decades (Alexis et al., Citation2010).
This review will briefly document the physicochemical, pharmacological properties of metformin, and also its mechanisms of action in type 2 diabetes and cancer. In addition, the studies with regard to microparticles and nanoparticles developed to enhance the bioavailability of metformin and eliminate/reduce the side effects, their use in the treatment of cancer will be summarized.
Physicochemical properties
Metformin hydrochloride (1,1-dimethylbiguanide hydrochloride; C4H11N5,HCl; ) is a white crystalline, odorless, hygroscopic and bitter in taste solid with a molecular weight of 165.625 and has two pKa values of 2.8 and 11.51. The aqueous solubility of metformin is 300 mg/mL within the pH range of 1.2–6.8. It is slightly soluble in alcohol, practically insoluble in acetone and dichloromethane (Sweetman, Citation2007; Raghuwanshi et al., Citation2010; Raparla & Talasila, Citation2012; Desai et al., Citation2014). Its melting point is between 222–226 °C. Metformin should be preserved in light-resistant and well-closed containers in room temperature (Food and Drug Administration, Citation2015).
Figure 1. Chemical structure of metformin hydrochloride (European Pharmacopeia, Citation2004).
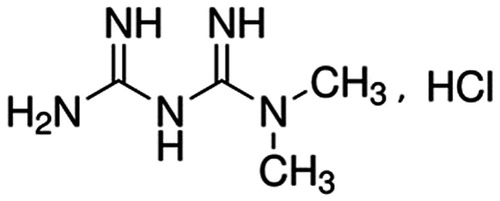
Metformin is a hydrophilic base, which has a cationic charge at physiological pH (cationic percentage at physiologic pH >99.9%) and thus, it has very limited passive diffusion across cell membranes (Graham et al., Citation2011). Because of its low permeability across the cell membranes and high aqueous solubility, metformin is classified as a Class 3 compound according to Biopharmaceutics Classification System (BCS) and Biopharmaceutics Drug Disposition Classification System (BDDCS) (Cheng et al., Citation2004; Sweetman, Citation2007; Shugarts & Benet, Citation2009; Graham et al., Citation2011). It is also a Class 2 compound according to the Salivary Excretion Classification System (SECS) (Idkaidek, Citation2014).
Pharmacokinetics properties
Metformin hydrochloride shows slow and incomplete absorption following oral administration. The absolute bioavailability of metformin after a 500 mg single dose is about 50–60%. Its bioavailability is reduced if taken with food (Sweetman, Citation2007). After oral administration of an immediate release metformin hydrochloride tablet (500 mg), the peak plasma concentration (1.0–1.6 mg/L) was reached within 3 h (Graham et al., Citation2011). Following oral administration of 1000 mg dose of immediate release-metformin tablet daily twice, the maximum plasma concentration (Cmax) was reported to be higher in type 2 diabetic patients with good renal function (2.09 mg/L) than those of healthy subjects (1.32 mg/L). On the other hand, total clearance was about 30% lower in the patients with diabetes in comparison to healthy subjects (881 mL/min versus 1265 mL/min) (Graham et al., Citation2011).
Idkaidek et al. (Citation2011) investigated the pharmacokinetic properties of metformin-extended release tablets (750 mg) under fasting and fed states (after high-fat breakfast) and compared the results with those of immediate release tablets (1000 mg) under fasting conditions. For extended release tablets, although bioavailability and time to reach Cmax were increased in the fed state as compared to the fasted state, there was no significant difference between fed and fasting conditions in regard to Cmax (794 ng/mL versus 832 ng/mL) and half-life (t1/2: 3.66 h versus 3.80 h) values. All pharmacokinetic parameters (Cmax: 1956 ng/mL, tmax: 2.58 h, AUC0→∞: 12 884 ngċh/mL), except half-life (t1/2: 3.39 h), determined for immediate release tablets were significanty different from the corresponding values (Cmax: 832 ng/mL, tmax: 4.3 h, AUC0→∞: 6167 ngċh/mL) obtained for the extended release formulation in fasting conditions. Similarly, food slightly increased the bioavailability of metformin from metformin hydrochloride (1000 mg) SR tablets in healthy Indian volunteers (Dsilva et al., Citation2013).
A bioequivalence study was performed on healthy Mexican volunteers for three metformin-containing formulations (a reference and two generic drug; 500 mg metformin tablets) after a single dose. Very similar Cmax (1163.5 ng/mL, 1184.6 ng/mL and 1167.8 ng/mL, reference and two generics, respectively) and AUC0–t values (6240.7 ngċh/mL, 6433.7 ngċh/mL and 6567.1 ngċh/mL, reference and two generics, respectively) were taken as an indication of bioequivalency of generics to the reference product by the authors (Montoya-Eguía et al., Citation2015)
The plasma concentration profile of metformin after oral administration complies with the “flip–flop” type because the rate of absorption is slower than that of elimination (Bhavesh et al., Citation2007; Garrison et al., Citation2015).
The binding of metformin to human plasma proteins is negligible after absorption. The mean apparent volume of distribution of metformin after single oral doses (850 mg) is 654 ± 358 L (Food and Drug Administration, Citation2015). Metformin accumulates in the kidney, salivary glands and walls of the small intestine. The plasma elimination half-life of metformin is about 2–6 h after oral doses. Metformin is renally eliminated by both filtration and active tubular secretion and excreted unchanged in the urine (Sweetman, Citation2007; Lipska et al., Citation2011).
Drug transporters for the absorption, distribution and elimination of many drugs have a key role and are important determinants of therapeutic and adverse drug effects (Morrissey et al., Citation2012). Metformin is a substrate of organic cation transporters 1, 2 and 3 (OCT1-3) predominantly expressed in liver and kidney, and its oral absorption, hepatic uptake and renal excretion occur largely via these transporters (Garrison et al., Citation2015). While OCT1 and possibly OCT3 play a role in the hepatic uptake of metformin, OCT2 expressed on the basolateral membrane of kidney tubular cells, is involved in the renal excretion of metformin. OCT1 and OCT2 may have a limited role in intestinal absorption of metformin. However, the dose-dependent intestinal absorption of metformin involves an active, saturable uptake process. Plasma membrane monoamine transporter (PMAT) is proton-activated organic cation transporter and transports many classic organic cations such as methyl phenylpyridinium and monoamine neurotransmitters. PMAT mRNA is expressed in many human tissues, including intestine, kidney, liver, brain and heart. PMAT expressed in human intestine may play a role in the intestinal absorption of metformin. Besides, PMAT expressed on the apical membrane of renal epithelial cells may be implicated in the renal reabsorption of metformin (Zhou et al., Citation2007; Gong et al., Citation2012). Additionally, metformin is a good substrate for human multidrug and toxin extrusion 1 (MATE1) and MATE2-K. MATE1 and MATE2-K expressed on the apical membrane of the renal proximal tubule cells, contribute to the renal excretion of metformin from the tubule cell to the lumen (Gong et al., Citation2012).
Pregnancy-related changes in renal filtration and net tubular transport affect the pharmacokinetics of metformin, and creatinine clearance may be used roughly for estimation of metformin renal clearance in pregnant patients (Eyal et al., Citation2010). Metformin is distributed into breast milk in small amounts, thus, infant exposure to metformin through the breast milk is low (Sweetman, Citation2007; Eyal et al., Citation2010). Metformin readily crosses the placenta and the fetus is exposed to metformin concentrations from negligible to as high as maternal concentrations (Eyal et al., Citation2010).
Pharmacological properties
Metformin and type 2 diabetes
Type 2 diabetes mellitus (DM) comprises of 90% of patient with diabetes all around the world is also called as noninsulin-dependent or adult onset (Harper et al., Citation2013; Jung et al., Citation2014; McCulloch & Munshi, Citation2014; World Health Organization, Citation2015). It is a metabolic disorder and characterized by insulin resistance in muscle, liver and adipose tissue, imbalances, abnormalities effecting multiple organ systems and ongoing decline in β-cell function resulting from the body’s ineffective use of insulin. As the diagnosis of type 2 diabetes is usually delayed, in 20–50% of patients with type 2 diabetes, the formation of microvascular and/or macrovascular complications are determined during the diagnosis (Harper et al., Citation2013). The prevalence of type 2 diabetes continues to increase because of excess body weight, physical inactivity and unhealty diet (Harper et al., Citation2013; Jung et al., Citation2014; World Health Organization, Citation2015). Therefore, in the treatment of patients with type 2 diabetes, healthy diet, regular physical activity and weight loss (progressive weight loss in patients causes an improvement of glycemia) are recommended in addition to pharmacological treatment (Pi-Sunyer, Citation2005; World Health Organization, Citation2015).
The pathophysiology of type 2 diabetes mellitus is characterized by a range of metabolic disorders. These include (a) endogenous glucose production rates are excessive in patients with type 2 diabetes due to insufficient suppression after eating, (b) in mild type 2 diabetes, after oral glucose uptake, blood glucose levels continue to increase for a longer period of time compared to nondiabetics, (c) the stimulation of hepatic glucose uptake induced by insulin is impaired in these patients, hepatic glycogen synthesis is mainly decreased, the extracellular glucose uptake is probably reduced, and there is insufficient activation of hepatic glucokinase (d) β-cell dysfunction and glucagon concentrations remain unsuppressed, (e) in patients with severe diabetes, there is more severe β-cell dysfunction and abnormal suppression of glucagon levels (Rizza, Citation2010; Thulé, Citation2012).
Although metformin has been used as an oral antihyperglycemic agent for the treatment of type 2 diabetes since 1950s; its mechanism of action has not been fully understood. However, recent studies demonstrated that metformin is effective on metabolic differences between normal and abnormal metabolic pathways (Grzybowska et al., Citation2011; Pernicova & Korbonits, Citation2014; Sankhyan & Pawar, Citation2013). The reductions in the hepatic glucose output and plasma-fasting insulin level, and also the reduction in insulin resistance contribute significantly to the glucose-lowering action of metformin (Zhou et al., Citation2001; Viollet et al., Citation2012; McCulloch, Citation2015).
The positive effects of metformin on the insulin receptor expression and tyrosine kinase activity lead to improvement in insulin sensitivity. Moreover, free fatty acid concentrations in serum are decreased because of its antilipolytic effect. Thus, the inhibition of gluconeogenesis occurs as result of reducing substrate availability for gluconeogenesis or changes in enzyme activities (Viollet et al., Citation2012; McCulloch, Citation2015). The activation of AMP-activated protein kinase (AMPK) enzyme is a major cellular regulator of lipid and glucose metabolism. AMPK participates in the phosphorylation and regulation of enzymes such as acetyl-CoA carboxylase (ACC), resulting in suppression of the expression of lipogenic enzymes, inducing the fatty acid oxidation, lowering cellular fatty acid synthesis in the liver and muscle, coordination of the changes in the hepatic lipid metabolism and increasing in glucose transport. It is activated by metformin and also in response to some metabolic stresses (e.g. ischemia, hypoxia) (Zhou et al., Citation2001; Park et al., Citation2002; Kim et al., Citation2011; Viollet et al., Citation2012; Su et al., Citation2014; McCulloch, Citation2015). Additionally, especially after eating, metformin increases insulin-mediated glucose utilization in peripheral tissues (muscle, liver, etc.) and decreases glucose absorption in the small intestine (Zhou et al., Citation2001; Viollet et al., Citation2012; McCulloch, Citation2015). It was also reported that metformin has an influence on the modulation of multiple components of the incretin axis; it increases the plasma level of glucagon-like peptide 1 and stimulates incretin receptor gene expression in islets cells via peroxisome proliferator-activated receptor PPAR-α. Furthermore, metformin stimulates the function of GLP-1 on β-cells, thereby increases insulin secretion (Kanazawa, Citation2011; Maida et al., Citation2011; Viollet et al., Citation2012).
Metformin and cancer
Recent studies showed that metformin might be used as an anticancer agent in the treatment of hyperinsulinemia-associated cancers, especially breast and colon cancers. The inhibitory effects of metformin on the proliferation of some cancer cells (prostate, breast, endometrial, ovarian, colon and glioma cells) have been demonstrated in the cell culture studies (Dowling et al., Citation2011; Kourelis & Siegel, Citation2012). Diabetic cancer patients treated with different antidiabetic agents have different survival rate. Metformin reduces cancer risk and cancer-related mortality in diabetic patient compared to the diabetic patients not treated with metformin (Dowling et al., Citation2011; Ganjali & Ganjali, Citation2013).
The anticancer effects of metformin are associated with its direct insulin-independent and indirect insulin-dependent actions. Its inhibitory effects on cancer cells occur through the activation of AMPK, Src tyrosine kinase, and mitogen-activated protein kinase (MAPK), the inhibition of mammalian target of rapamycin signaling and protein synthesis and the reduction of epidermal growth factor receptor (EGFR). In addition, metformin may target cancer-initiating cells. As increased insulin levels and obesity are adverse prognostic factors for some cancer types, the lowering effects of metformin on insulin levels may play a major role in its anticancer activity (Dowling et al., Citation2011).
Zhang et al. (Citation2014) investigated the effects of metformin on epithelial–mesenchymal transition (EMT) that has an important role in cancer progression, metastasis in prostate cancer cells and microRNA (miRNA)-based mechanisms. It was reported that metformin inhibits EMT, downregulates SOX4 (a 47 kDa protein overexpressed in prostate cancer) and upregulates tumor suppressor miR30a.
In breast cancer cells, metformin effects signaling in the AKT/mTOR pathway and the activation of AMPK. Daily use of 500 mg metformin was reported to be useful for inhibiting the breast cancer cells (Ganjali & Ganjali, Citation2013). Similar to the motility, the growth and aggression of breast cancer cells are promoted by high glucose levels, higher glucose levels reduce the effect of metformin on breast cancer (Wahdan-Alaswad et al., Citation2013).
Metformin causes the accumulation of reactive oxygen species (ROS) leading to cell death. Dichloroacetate is a widely used drug for the treatment of lactic acidosis by means of inhibition of pyruvate dehydrogenase kinase. Combination of dichloroacetate with metformin was reported to synergistically induce caspase-dependent apoptosis involving oxidative damage (Haugrud et al., Citation2014).
Orecchioni et al. (Citation2015) studied the effects of metformin and phenformin on breast cancer using in vitro and in vivo models, and found that phenformin was more effective than metformin in both models. Both drugs were reported to induce apoptosis of breast cancer and the human white adipose tissue (WAT) endothelial cells through the activation of AMPK and inhibition of Complex 1 of the respiratory chain in the in vitro model. WAT contains progenitors with cooperative roles in breast cancer angiogenesis, local and metastatic progression. Also, in the in vivo model, these drugs were found to inhibit angiogenesis as a result of reduction in tumor microvessel density and increase in pimonidazole binding of progenitors.
Long-term administration of metformin in the treatment of diabetes leads to a reduction in the incidence of colorectal cancer (Ioannou & Boyko, Citation2011). Nangia-Makker et al. (Citation2014) investigated the effect of metformin combination with 5-fluorouracil and oxaliplatin on the survival of chemoresistant colon cancer cells. The combination of metformin and oxaliplatin shows synergistic effect and induce chemoresistant colon cancer cell death and inhibits cell proliferation, migration. Consequently, the authors suggested that metformin administration with conventional chemotherapeutics may be effective treatment regimen for recurring colorectal cancer.
Metformin and polycystic ovary syndrome
Polycystic ovary syndrome (PCOS) is a common endocrine disorder effecting the population of reproductive-aged women. PCOS is promoted by hormonal disturbances (hyperandrogenism and insulin resistance) and there is a strong correlation between hyperandrogenism and hyperinsulinemia. Metformin has been reported to increase insulin sensitivity, reduce insulin level and improve androgen concentrations and menstrual cycles in women presenting insulin resistance and dysglicemia. Thus, significant reductions in serum androgen levels are seen after metformin treatment (Mayer et al., Citation2015; Naderpoor et al., Citation2015; Spritzer et al., Citation2015).
Adverse reactions
Most commonly observed adverse effects associated with metformin are nausea, mild anorexia, a metallic taste in the mouth, abdominal discomfort and soft bowel movements or diarrhea. The absorption of vitamin B12 may be impaired by metformin. Skin reactions are seen rarely. Metformin can cause lactic acidosis in patients with normal renal and hepatic functions. Sometimes lactic acidosis can be fatal. The drug interactions exist between metformin and blood-glucose-lowering drugs. Hypoglycemia is rare when a biguanide is given alone, but it may ocur when metformin combined with other drugs, such as sulfonylureas and insulin. Cimetidine may lower the renal clearance of metformin due to competitive inhibition of renal tubular secretion of metformin (Sweetman, Citation2007; McCulloch, Citation2015).
Metformin is not metabolized. Therefore, there is no metabolic interaction with metformin (Scheen, Citation2005). However, OCT system is very important in terms of metformin interaction with other drugs. Flory et al. (Citation2015) reported that metformin is taken up into hepatocytes by OCT system. Although OCTs are inhibited by proton pump inhibitors (PPIs), there is no evidence of a deleterious interaction between PPIs and metformin (Flory et al., Citation2015). Vandetanib, an OCT substrate, is a selective inhibitor of vascular endothelial growth factor receptor and epidermal growth factor receptor. Coadministration of vandetanib and metformin demonstrated that Cmax and AUC values of metformin increased by 74% and 50%, respectively, and its renal clearance decreased by 52% (Johansson et al., Citation2014). In addition, hepatocyte uptake of metformin via OCT1 is strongly inhibited by verapamil; as a result, glucose-lowering effect of metformin is decreased (Cho et al., Citation2014).
In aged rats, rOCT2 (expressed in the renal basolateral membrane) protein expression is significantly decreased. Both metformin and atenolol are eliminated by OCT2 (OCT2/SLC22A2). Therefore, when metformin and atenolol were coadministered, atenolol competitively affects the renal excretion of metformin in aged rats (Ren et al., Citation2015).
Metformin-loaded microparticles and nanoparticles
Drug delivery systems (microparticles, nanoparticles, liposomes, niosomes, etc.) are very useful systems to overcome the difficulties associated with conventional dosage forms. These systems have lots of advantages compared to the traditional dosage forms such as effective protection of drugs against degradation (enzymatic), reduction of drug’s side effects and its repeated doses, increasing patient comfort and compliance, control of drug release or the site of drug release and increasing the relative bioavailability of drugs (Varde & Pack, Citation2004; Ravi Kumar, Citation2000; Satheesh Madhav & Kala, Citation2011; Hasan et al., Citation2013; Momoh et al., Citation2013).
Microsized polymeric particles (1–1000 micron) have an important role as drug delivery systems aimed to provide the sustained or controlled drug relase for a longer periods of time, to improve bioavailability of drugs, to protect drug from the environment and reduce its side effects (Pavan Kumar et al., Citation2011; Satheesh Madhav & Kala, Citation2011).
There are two types of microparticulate formulations, namely microspheres and microcapsules (as subcategory). Microspheres are referred to as spherical micrometric matrix systems. Microcapsules are reservoir systems containing core substances (solid, liguid or gas) (Singh et al., Citation2010; Patel et al., Citation2011).
Several papers are available in the literature in regard to metformin HCl-loaded microcapsules. One of these is involved in mucoadhesive microcapsules developed by Kumar et al. (Citation2011) using ionotropic gelation and emulsification ionotropic gelation methods. Sodium alginate and Gum Karaya (1:1 w/w) were used as the coating material in the preparation of microcapsules. The results of this study revealed that approximately 80% of microcapsules were spherical with a size range of 939–1204 micron and completely covered with the coating polymers. The microcapsules showed free flow (angle of repose ≤24; Hausner ratio <1.3 and Carr's index values <24). The encapsulation efficiency values of all formulations were in the range of 73–86%. The DSC thermograms obtained for pure drug and microcapsule formulations demonstrated that there was no interaction between drug and polymers. The preparation methods and core:coat ratio (1:1;1:1.5;1:2) influenced the release properties of metformin HCl from microcapsules. When the core:coat ratio increased to 1:2, slower drug release was obtained at pH 1.2 medium for both methods. Drug release studies indicated that microcapsules with controlled release properties were prepared by emulsification ionotropic gelation method and also they have good mucoadhesive properties.
Nath et al. (Citation2009) developed floating microcapsules for metfomin HCl using different polymers (Eudragit RL 100 and Cellulose acetate butyrate with differrent permeability properties and molecular weight of 150 000 and 16 000, respectively) by nonaqueus emulsion-solvent evaporation method. SEM analysis showed that all microcapsule formulations were spherical in shape. The encapsulation efficiency was the highest (90% and 92% at stirring speed of 1000 rpm and 1800 rpm, respectively) for microcapsules prepared using Eudragit RL 100:cellulose acetate butyrate (1:1 w/w of ratio) mixture compared to the microcapsule formulations prepared with one polymer only. This observation was attributed to the higher relative viscosity of organic phase containing polymers mixture, and the formation of most stable emulsion and followed by the most suitable structure of microcapsules. Particle sizes of microcapsules were influenced by the stirring speed. For stirring speed of 1000 rpm, the mean particle sizes of the microparticles prepared using Eudragit RL, cellulose acetate butyrate and polymers mixture (1:1) were reported as 371 micron, 534 micron and 710 micron, respectively. When stirring speed increased to 1800 rpm, the mean size of microcapsules prepared using the mixture of polymers (1:1) was decreased to 381 micron. Maximum floating behaivour in 0.1 M HCl was observed for Eudragit RL 100 microcapsules (about 10 h), whereas it was minimum for cellulose acetate butyrate microcapsules. The floating ability of microcapsules prepared from polymers mixture was higher than those of cellulose acetate butyrate microcapsules. The in vitro drug release from Eudragit RL 100 microcapsules was faster (81–83% and 85–89% at 8–10 h at pH 1.2 and pH 6.8, respectively) than those of cellulose acetate butyrate microcapsules (59–67% and 67–71% at 8–10 h at pH 1.2 and pH 6.8, respectively). Slower drug release from the cellulose acetate butyrate microcapsules was attributed to the thicker polymeric surface of the microparticles. As Eudragit RL 100 in polymer mixture was more permeable due to the higher amount of quaternary ammonium groups, the drug release from the microcapsules prepared from polymer mixture was reported to be faster (77% and 81% at 10 h at pH 1.2 and pH 6.8, respectively) than the release of cellulose acetate butyrate microcapsules.
The Wurster-fluidized bed was used for preparation of sustained release metformin HCl microcapsules by Cao et al. (Citation2014). By controlling atomization air pressure and coating temperature, microcapsules were prepared without aggregation. The results of the study revealed that the mean size of microparticles was 213 micron and contained 23% of initial load of metformin HCl. Also, sustained release of metformin HCl from microcapsules was demonstrated.
In another study, some polymers (Eudragit S100, cellulose acetate ethylcellulose) were used to prepare metformin HCl-loaded microcapsules. Preparation of microparticles using oil in oil (o/o) solvent evaporation technique resulted in a spherical microparticle with continuous coating and no aggregation (Shivhare et al., Citation2009).
Choudhury et al. (Citation2008) prepared cellulose acetate microspheres for gastric retention by emulsion–solvent evaporation method. When different polymer:drug concentration ratios (2:1 and 4:1) were used for the preparation of microspheres, the mean particle sizes and buoyancy percentage were found to be 7 and 18 microns, and ∼56% and ∼88%, respectively. Based on the results, it was concluded that the polymer concentration was important for particle size of microspheres, and particle size increased with an increase in polymer concentration. The entrapment efficiencies of microspheres were slightly increased with an increase in polymer concentration (87% and 89% for polymer:drug concentration ratios of 2:1 to 4:1). On the other hand, with an increase in the polymer:drug concentration ratio from 2:1 to 4:1, the in vitro cumulative drug release in 0.1 M HCl for 12 h was decreased from 87% to 49%. This observation was attributed to the increasing diffusional path length with an increase in polymer concentration and also in the density of polymer matrix. The in vivo efficiency of microspheres (equivalent to 60 mg/kg body weight of metformin HCl) was investigated in healthy male albino mice after oral administration. The results revealed that plasma glucose levels declined rapidly (at 30 min) and returned to its first level within 2 h with the administration of pure drug. On the other hand, it declined slowly and reached to the maximum reduction 3.5 h after microspheres administration.
Choudhury & Kar (Citation2009) used emulsion–solvent evaporation (ESE) and nonsolvent (petroleum ether) addition methods (NSA) for the preparation of metformin HCl-containing ethyl cellulose microspheres with controlled release properties. At 800 rpm, the maximum number of microspheres was prepared. When the stirring speed increased (1000 rpm) or decreased, irregularly shaped microspheres and large-sized microspheres (≥500 micron), respectively, were produced. It was reported that amount of emulsifier (Span 80), the addition rate of nonsolvent, and drug/polymer ratio affect particle size and shape of the microspheres. When Span 80 (up to 1%) and polymer concentration ratio (above 1%) increased, smaller microspheres (200–400 micron) and microspheres with smoother surfaces were produced, respectively. The % yield values of microspheres prepared by ESE and NSA methods were about 72–87% and 71–90%, respectively. When stirring speed was decreased to 800 rpm, the entrapment efficiency (EE%) values of microspheres were increased (∼65–78% for ESE method and ∼68–86% for NSA method). The results of the in vitro release studies carried out in pH 1.2 buffer without pepsin, demonstrated that high polymer concentration (1:2) reduced the drug release from the microspheres because of increased thickness of the polymer matrix. The drug release from microspheres prepared by NSA method was sustained for about 10 h. On the other hand, about 90% of drug was released from microspheres prepared by ESE method within ∼8 h. When pure drug and microsphere formulations were administered orally (equivalent to 60 mg/kg body weight of metformin HCl) to normal and hyperglycemic male albino mice, microspheres caused slower reduction in plasma glucose level. The maximum reduction in glucose level was observed 30 min, 3.5 h and 4 h after the administration of pure drug, microspheres prepared by ESE and NSA methods, respectively. Based on the results, authors concluded that hypoglycemic effect of drug could be sustained for about 10 h with microspheres prepared by two methods.
Another group formulated metformin HCl-containing pectin microspheres by w/o emulsion solvent evaporation method using different ratios of drug:pectin (1:0.5–1:4), and also with different polymers (ethyl cellulose, HPMC and Acrycoat S100; drug:polymer ratio 1:1). The particle sizes of all microsphere formulations were in the range of 35–84 micron with a narrow distribution. When low and high polymer concentrations (drug:polymer 1:1 and 1:4, respectively) were used, the particle size increased from ∼35 to 71 micron, and the drug entrapment efficiency from about 27% to 60%. The particle size and entrapment efficiency values of microspheres made of ethyl cellulose, HPMC and Acrycoat S100 were 39 micron, 42 micron and 48 micron, and about 42%, 30% and 33%, respectively. The cumulative drug release from the microspheres prepared using different pectin ratio and different polymers was in range of ∼95–98% and ∼46 (for Acrycoat S100) −95% (for ethyl cellulose) within 9 h in pH 6.8 buffer, respectively. The authors reported that pectin microspheres showed better controlled release of metformin HCl compared to microspheres made of the other polymers. When the effects of metformin HCl-containing pectin microspheres and pure drug (equivalent 25 mg/kg body weight) were evaluated on diabetic rats after oral administration, it was found that microspheres continuously decreased the blood glucose level for about 5 h (from 297.5 to 173 mg/dL) compared to that of pure drug (maximum decrease obtained at 1 h, from 298.7 to 134.4 mg/dL) (Banerjee et al., Citation2012).
Wang et al. (Citation2013) extracted a crude polysaccharide from the Mactra veneriformis (a marine clam; it is used as a seafood and also in traditional Chinese medicine). Pure polysaccharide (MVPS) was obtained after the removal of protein impurities by dialysis process. The authors prepared metformin HCl-loaded microspheres with MVPS and also composite microspheres with MVPS and additive polymers (PVA-124, PEG-6000 and chitosan) by spray drying method. The production yield of microsphere formulations was in the range of ∼46–70%. SEM images of the microspheres demonstrated that MVPS microspheres were about spherical in shape with polymer aggregation, PVA-MVPS and PEG-MVPS microspheres appeared like shriveled microcapsules. However, chitosan-MVPS microsphere has a smooth and spherical shape due to the change of viscosity of polymer feeding solution. The particle size values of PVA-MVPS, PEG-MVPS and chitosan-MVPS microspheres were 5.5 micron, 6.8 micron and 4.2 micron, respectively. The particle size of MVPS microspheres was not measured due to the intense aggregation. Thus, chitosan-MVPS microsphere was selected for further studies. The encapsulation efficiency of the microspheres was determined as 89%. About 65% and 90% of metformin HCl were released within 25 and 90 min, respectively. The authors reported that chitosan/MVPS microspheres loaded with drug can be suitable for nasal application because of its particle sizes (<10 micron).
Metformin HCl-loaded bioadhesive chitosan microparticles for oromucosal drug administration were prepared by spray-drying method. The production yield and mean particle size of chitosan microparticles were 88% and about 6 micron, respectively. The bioadhesion of chitosan microparticles to porcine buccal mucosa (does not contain mucin on mucosa) was investigated by ex vivo flow retention model. The adherence of microparticles to buccal mucosa was attributed to the combination of dehydration of water from the mucosa and swelling. A chitosan–water gel forms at the contact stage, electrostatic and hydrophobic interactions occur between positively charged chitosan and negatively charged epithelial surface-bound proteins at the consolidation stage. It was also reported that the irrigation media affects the bioadhesive properties of microparticles. Irrigation media (containing polymer or mucin) with high viscosity supplies a higher amount of water, thereby increasing water uptake of microparticles followed by swelling and formation of electrostatic and hydrophobic interactions, and enhancement of bioadhesion strength (Madsen et al., Citation2013).
Floating ethyl cellulose microspheres containing metformin HCl was prepared by emulsion solvent evaporation method using different drug:polymer concentration ratio (1:1-1:6), and then, its pharmacokinetic and pharmacodynamic properties were evaluated (Pandit et al., Citation2013). The production yield values of all microsphere formulations were higher than 85%, and the entrapment efficiency values were in the range of 45–82%. The highest production yield (89.6%), entrapment efficiency (about 82%) and buoyancy (99.9%) values were obtained for the microspheres prepared using 1:2 of drug:polymer ratio. The mean particle size of microsphere for this drug:polymer ratio was about 10 microns, whereas others were in the range of 9.43–17.66 micron. The Hausner’s ratio and compressibility (%) values of microsphere formulations were less than 1.25% and 10%, respectively. The pharmacodynamic effect and pharmacokinetic properties of microspheres (drug:polymer ratio: 1:2) were determined in diabetic and healthy male Wistar rats, respectively. The biological t1/2 for microsphere formulation was >14 h. AUCtotal values were 59 µg.h/mL, 16.6 µg.h/mL and 29 µg.h/mL, for microsphere, pure drug and commercial formulation, respectively. When the microsphere formulation was used, the relative bioavailability of metformin HCl increased about 3.5-fold compared to that of pure drug. After administration of microsphere formulation, pure drug and commercial formulation to the diabetic rats, the blood glucose levels were found to be in the range of 125–140 mg/dL, 180–185 mg/dL and 160–170 mg/dL, respectively. These results indicate that microsphere formulation significantly decreases the blood glucose levels in comparison to the pure drug and commercial product.
Nanoparticles are defined as submicronic (1–1000 nm) colloidal systems (Brigger et al., Citation2002). Nanoparticles have been investigated as the delivery systems for a wide number of drugs. They have the advantages of high stability in lyophilized or appropriate formulation, feasibility for the incorporation of both hydrophobic and hydrophilic active substances, high carrier capacity of many drug molecules and feasibility for various routes of administration (e.g. oral, nasal, parenteral) (Galindo-Rodriguez et al., Citation2005; Gelperina et al., Citation2005). Nanoparticles are especially unique approaches for cancer treatment (Alexis et al., Citation2010). In the literature, various metformin HCl-containing nanoparticle formulations are available. These formulations differ mainly with respect to polymers used and method of preparation.
Cetin et al. (Citation2013) prepared and characterized metformin HCl-loaded nanoparticle formulations by the nanoprecipitation method using both a single polymer (Eudragit®RSPO) and a polymer mixture (Eudragit/PLGA). SEM images clearly demonstrated that all nanoparticles were spherical in shape and have a smooth surface. The mean particle size ranged from 268.8 to 288 nm with zeta potential values of 9.72 to 10.1 mV. The highest encapsulation efficiency values were observed for Eudragit® RSPO (300 mg) nanoparticles. All formulations showed highly reproducible drug release profiles and the in vitro drug release in phosphate buffer (pH = 6.8) ranged from 92 to 100% in 12 h.
In another study, metformin HCl-loaded CS-PLGA nanoparticles were prepared using modified nanoprecipitation method and then evaluated. The particle sizes of blank nanoparticles, 50 mg of metformin HCl-loaded nanoparticles and 75 mg of metformin HCl-loaded nanoparticles were ranged from 507 to 516 nm with surface charges of 23–32 mV. Low encapsulation efficiency was observed for both nanoparticle formulations. Nanoparticle formulations showed highly reproducible drug release profiles. About 20% of metformin HCl was released within 30 min and approximately 98% of the loaded metformin HCl was released within 144 h in phosphate buffer (pH 6.8). However, no statistically significant difference was noted between the in vitro release profiles of nanoparticle formulations containing metformin HCl (Gundogdu & Cetin, Citation2014).
When coacervation method was used for the preparation of metfomin-containing BSA nanoparticles, spherical nanoparticles with a mean size of 97 nm with narrow distribution were obtained. It was found that drug-loaded nanoparticles were more toxic on proliferation of pancreatic carcinoma cells when compared with pure metformin (Jose et al., Citation2015).
In another study, metformin-containing nanoparticles with a mean size <300 nm, prepared by solvent evaporation method using different polymers (polymethyl methacrylate: PMMA and PLGA) were examined for their toxicological properties. Haemolytic effects of nanoparticles were less than 5%, indicating that they were haemocompatible. When the toxic effects of nanoparticles were evaluated 30 days after administration to rats, the histopathologic analysis of various organs such as heart, lung, liver and kidney showed that metformin-loaded PMMA and PLGA nanoparticles did not seriously damage the organs and also had no toxic effects in rats (Lekshmi & Reddy, Citation2012).
Metformin HCl-loaded O-carboxymethyl chitosan nanoparticles were prepared by ionic-gelation method for the delivery of drug to pancreatic cancer cells. The average size of spherical nanoparticles was 230 ± 50 nm. They have negative surface charge (−18.32 mV at pH 7.4) due to the unreacted carboxyl group of O-carboxymethyl chitosan. In vitro release studies revealed that 90% of metformin HCl released at pH 4.4 within 1 h, whereas 50% and 72% of metformin was released from the nanoparticles at pH 7.4 within 10 h and 70 h, respectively. This observation indicates that the metformin release from nanoparticles is sensitive to the pH of the medium. Although pure metformin and metformin-loaded nanoparticles have no toxic effect on normal cells (L929), metformin HCl and metformin HCl-loaded nanoparticles showed more toxic effect on pancreatic cancer cells (MiaPaCa-2) as compared to blank nanoparticles. Nanoparticles were taken up nonspecifically by normal and pancreatic cancer cells. Degree of hemolysis caused by drug loaded and blank nanoparticles was less than 5% (Snima et al., Citation2012).
Snima et al. (Citation2014) also evaluated the O-carboxymethyl chitosan nanoparticles for pancreatic cancer therapy by means of in vitro (migration assay, clonogenic assay, cell cycle analysis and qRT-PCR analysis) and in vivo (biodistribution studies) studies. In cell migration study, the degrees of wound closure were 13.5%, 7% and 12% for MiaPaCa-2 cells treated with medium, blank-nanoparticles and metformin-loaded nanoparticles after 8 h of incubation, respectively. The corresponding values were 66%, 54.5% and 63% after 30 h of incubation. The clonogenic assay carried out using 1000 viable MiaPaCa-2 cells and demonstrated that metformin-loaded nanoparticles (the average number of colonies: 785) reduced colony formation ability of the cancer cells as compared to blank nanoparticles (the average number of colonies: 1008) and untreated cells (the average number of colonies: 1202). Metformin-loaded nanoparticles had only minor effect on cell cycle progression in MiaPaCa-2 cells. Additionally, it was shown by qRT-PCR analysis that the expression of mRNAs related to cell cycle regulation (p21) and metastasis (MMP-9 and Vannin-1) were decreased in MiaPaCa-2 cells treated with metformin-loaded nanoparticles (equivalent to 3.7 mM of metformin). In vivo biodistribution study showed normal biodistribution pattern without toxicity following intravenous administration of metformin-loaded nanoparticles
Conclusion
Metformin is the most widely prescribed oral antihyperglycemic agent for the treatment of type 2 diabetes. However, it has a slow and incomplete absorption following oral administration and repeated applications of high doses of metformin (as immediate release formulations) are needed for an effective treatment due to its short biological half-life. Furthermore, recent studies demonstrated that metformin has potential antitumorigenic effects on different cancer types, such as breast and colon cancers. Therefore, metformin is an ideal candidate for cancer therapy due to its clinical safety, low cost and adequately known pharmacodynamic profile. Drug delivery systems are very useful systems to overcome the difficulties associated with conventional dosage forms. Therefore, the development of drug delivery systems (e.g. microparticles, nanoparticles) strategies for metformin might be useful not only to improve its bioavailability, to reduce the dosing frequency, to decrease gastrointestinal side effects and toxicity and also to be helpful for the effective use of metformin in cancer treatment.
Declaration of interest
The authors declare no conflicts of interest.
References
- Adikwu MU, Yoshikawa Y, Takada K. (2003). Bioadhesive delivery of metformin using prosopis gum with antidiabetic potential. Biol Pharm Bull 26:662–6
- Alexis F, Pridgen EM, Langer R, et al. (2010). Nanoparticle technologies for cancer therapy. Handb Exp Pharmacol 197:55–86
- Amorim MJ, Ferreira JP. (2001). Microparticles for delivering therapeutic peptides and proteins to the lumen of the small intestine. Eur J Pharm Biopharm 52:39–44
- Banerjee P, Deb J, Roy A, et al. (2012). Fabrication and development of pectin microsphere of metformin hydrochloride. ISRN Pharm 2012:230621. doi: 10.5402/2012/230621
- Basavaraj S, Betageri GV. (2014). Can formulation and drug delivery reduce attrition during drug discovery and development-review of feasibility, benefits and challenges. Acta Pharm Sin B 4:3–17
- Bhavesh D, Chetan G, Bhat KM, et al. (2007). Estimation and pharmacokinetics of metformin in human volunteers. Indian J Pharm Educ Res 41:135–9
- Boldhane SP, Kuchekar BS. (2009). Gastroretentive drug delivery of metformin hydrochloride: formulation and in vitro evaluation using 3(2) full factorial design. Curr Drug Deliv 6:477–85
- Brigger I, Dubernet C, Couvreur P. (2002). Nanoparticles in cancer therapy and diagnosis. Adv Drug Deliv Rev 54:631–51
- Cao J, Liu H, Pan W, et al. (2014). The preparation of the sustained release metformin hydrochloride microcapsules by the Wurster fluidized bed. Pak J Pharm Sci 27:779–84
- Cetin M, Atila A, Sahin S, et al. (2013). Preparation and characterization of metformin hydrochloride loaded-Eudragit®RSPO and Eudragit®RSPO/PLGA nanoparticles. Pharm Dev Technol 18:570–6
- Cheng CL, Yu LX, Lee HL, et al. (2004). Biowaiver extension potential to BCS class III high solubility-low permeability drugs: bridging evidence for metformin immediate-release tablet. Eur J Pharm Sci 22:297–304
- Cho SK, Kim CO, Park ES, et al. (2014). Verapamil decreases the glucose-lowering effect of metformin in healthy volunteers. Br J Clin Pharmacol 78:1426–32
- Choudhury PK, Kar M, Chauhan CS. (2008). Cellulose acetate microspheres as floating depot systems to increase gastric retention of antidiabetic drug: formulation, characterization and in vitro-in vivo evaluation. Drug Dev Ind Pharm 34:349–54
- Choudhury PK, Kar M. (2009). Controlled release metformin hydrochloride microspheres of ethyl cellulose prepared by different methods and study on the polymer affected parameters. J Microencapsul 26:46–53
- Corti G, Cirri M, Maestrelli F, et al. (2008). Sustained-release matrix tablets of metformin hydrochloride in combination with triacetyl-β-cyclodextrin. Eur J Pharm Biopharm 68:303–9
- Desai D, Wong B, Huang Y, et al. (2014). Surfactant-mediated dissolution of metformin hydrochloride tablets: wetting effects versus ion pairs diffusivity. J Pharm Sci 103:920–6
- Dowling RJ, Goodwin PJ, Stambolic V. (2011). Understanding the benefit of metformin use in cancer treatment. BMC Med 9:33
- Dsilva LC, Palmer J, Sudershan V, et al. (2013). Effect of food on the absorption of metformin from sustained release metformin hydrochloride formulations in healthy Indian volunteers. Asian J Pharm Clin Res 6:95–9
- European Pharmacopoeia. (2004). 2005 with Supplements 5.1 and 5.2. 5th ed. Main Volume 5.0. Strasbourg: Council of Europe, 2003 p
- Eyal S, Easterling TR, Carr D, et al. (2010). Pharmacokinetics of metformin during pregnancy. Drug Metab Dispos 38:833–40
- Flory J, Haynes K, Leonard CE, et al. (2015). Proton pump inhibitors do not impair the effectiveness of metformin in diabetic patients. Br J Clin Pharmacol 79:330–6
- Food and Drug Administration. Metformin hydrochloride tablet. Retrieved March 28, 2015. Available at: http://www.fda.gov/ohrms/dockets/dailys/02/May02/053102/800471e6.pdf, 20.03.2015 [last accessed 11 Sep 2015]
- Galindo-Rodriguez SA, Allemann E, Fessi H, et al. (2005). Polymeric nanoparticles for oral delivery of drugs and vaccines: a critical evaluation of in vivo studies. Crit Rev Ther Drug Carrier Syst 22:419–64
- Ganjali M, Ganjali H. (2013). Anticancer effect of metformin, an anti diabetic drug, on breast Cancer Cells. J Nov Appl Sci 2:796–801
- Garrison KL, Sahin S, Benet LZ. (2015). Few drugs display flip-flop pharmacokinetics and these are primarily associated with classes 3 and 4 of the BDDCS. J Pharm Sci 104:3229–35
- Gelperina S, Kisich K, Iseman MD, et al. (2005). The potential advantages of nanoparticle drug delivery systems in chemotherapy of tuberculosis. Am J Respir Crit Care Med 172:1487–90
- Gong L, Goswami S, Giacomini KM, et al. (2012). Metformin pathways: pharmacokinetics and pharmacodynamics. Pharmacogenet Genomics 22:820–7
- Graham GG, Punt J, Arora M, et al. (2011). Clinical pharmacokinetics of metformin. Clin Pharmacokinet 50:81–98
- Grzybowska M, Bober J, Olszewska M. (2011). Metformin-mechanisms of action and use for the treatment of type 2 diabetes mellitus. Postepy Hig Med Dosw (Online) 65:277–85
- Gundogdu N, Cetin M. (2014). Chitosan-poly (lactide-co-glycolide) (CS-PLGA) nanoparticles containing metformin HCl: preparation and in vitro evaluation. Pak J Pharm Sci 27:1923–9
- Harper W, Clement M, Goldenberg R, et al. (2013). Canadian diabetes association, clinical practice guidelines for the prevention and management of diabetes in Canada: pharmacologic management of type 2 diabetes. Can J Diabetes 37:S61–8
- Hasan AA, Madkor H, Wageh S. (2013). Formulation and evaluation of metformin hydrochloride-loaded niosomes as controlled release drug delivery system. Drug Deliv 20:120–6
- Haugrud AB, Zhuang Y, Coppock JD, et al. (2014). Dichloroacetate enhances apoptotic cell death via oxidative damage and attenuates lactate production in metformin-treated breast cancer cells. Breast Cancer Res Treat 147:539–50
- Idkaidek N, Arafat T, Melhim M, et al. (2011). Metformin IR versus XR pharmacokinetics in humans. J Bioequiv Availab 3:233–5
- Idkaidek NM. (2014). Interplay of biopharmaceutics, biopharmaceutics drug disposition and salivary excretion classification systems. Saudi Pharm J 22:79–81
- Ioannou GN, Boyko EJ. (2011). Metformin and colorectal cancer risk in diabetic patients. Diabetes Care 34:2336–7
- Johansson S, Read J, Oliver S, et al. (2014). Pharmacokinetic evaluations of the co-administrations of vandetanib and metformin, digoxin, midazolam, omeprazole or ranitidine. Clin Pharmacokinet 53:837–47
- Jose P, Sundar K, Anjali CH, et al. (2015). Metformin-loaded BSA nanoparticles in cancer therapy: a new perspective for an old antidiabetic drug. Cell Biochem Biophys 71:627–36
- Jung SH, Chae JW, Song BJ, et al. (2014). Bioequivalence comparison of two formulations of fixed-dose combination glimepiride/metformin (2/500 mg) tablets in healthy volunteers. Iran J Pharm Res 13:365–71
- Kanazawa I. (2011). A novel attribute of insulin secretion via incretin axis by metformin drug. Int J Endocriol Metab 9:420–1
- Kim HG, Hien TT, Han EH, et al. (2011). Metformin inhibits p-glycoprotein expression via the NF-κB pathway and CRE transcriptional activity through AMPK activation. Br J Pharmacol 162:1096–108
- Kourelis TV, Siegel RD. (2012). Metformin and cancer: new applications for an old drug. Med Oncol 29:1314–27
- Kumar A, Balakrishna T, Rajiv J, et al. (2011). Formulation and evaluation of mucoadhesive microcapsules of metformin HCl with gum karaya. Int J Pharm Pharm Sci 3:150–5
- Lekshmi UM, Reddy PN. (2012). Preliminary toxicological report of metformin hydrochloride loaded polymeric nanoparticles. Toxicol Int 19:267–72
- Lipska KJ, Bailey CJ, Inzucchi SE. (2011). Use of metformin in the setting of mild-to-moderate renal insufficiency. Diabetes Care 34:1431–7
- Madsen KD, Sander C, Baldursdottir S, et al. (2013). Development of an ex vivo retention model simulating bioadhesion in the oral cavity using human saliva and physiologically relevant irrigation media. Int J Pharm 448:373–81
- Maida A, Lamont BJ, Cao X, et al. (2011). Metformin regulates the incretin receptor axis via a pathway dependent on peroxisome proliferator-activated receptor-α in mice. Diabetologia 54:339–49
- Marathe PH, Wen Y, Norton J, et al. (2000). Effect of altered gastric emptying and gastrointestinal motility on metformin absorption. Br J Clin Pharmacol 50:325–32
- Mayer SB, Evans WS, Nestler JE. (2015). Polycystic ovary syndrome and insulin: our understanding in the past, present and future. Womens Health (Lond Engl) 11:137–49
- McCulloch DK. (2015). Metformin in the treatment of adults with type 2 diabetes mellitus. Available from: http://www.uptodate.com/contents/metformin-in-the-treatment-of-adults-with-type-2-diabetes-mellitus# [last accessed 11 Sep 2015]
- McCulloch DK, Munshi M. (2014). Treatment of type 2 diabetes mellitus in the older patient, Available from: http://www.uptodate.com/contents/treatment-of-type-2-diabetes-mellitus-in-the-older-patient [last accessed 11 Sep 2015]
- Momoh MA, Kenechukwu FC, Attama AA. (2013). Formulation and evaluation of novel solid lipid microparticles as a sustained release system for the delivery of metformin hydrochloride. Drug Deliv 20:102–11
- Montoya-Eguía SL, Garza-Ocañas L, Badillo-Castañeda CT, et al. (2015). Study among 3 metformin formulations in healthy Mexican volunteers: a single-dose, randomized, open-label, 3-period crossover study. Curr Ther Res 77:18–23
- Morrissey KM, Wen CC, Johns SJ, et al. (2012). The UCSF-FDA transportal: a public drug transporter database. Clin Pharmacol Ther 92:545–6
- Murphy C, Pillay V, Choonara YE, et al. (2012). Optimization of a dual mechanism gastrofloatable and gastroadhesive delivery system for narrow absorption window drugs. AAPS Pharm Sci Tech 13:1–15
- Naderpoor N, Shorakae S, Joham A, et al. (2015). Obesity and polycystic ovary syndrome. Minerva Endocrinol 40:37–51
- Nangia-Makker P, Yu Y, Vasudevan A, et al. (2014). Metformin: a potential therapeutic agent for recurrent colon cancer. PLoS One 9:e84369
- Nath B, Nath LK, Mazumdar B, et al. (2009). Design and development of metformin HCl floating microcapsules using two polymers of different permeability characteristics. IJPSN 2:627–37
- Orecchioni S, Reggiani F, Talarico G, et al. (2015). The biguanides metformin and phenformin inhibit angiogenesis, local and metastatic growth of breast cancer by targeting both neoplastic and microenvironment cells. Int J Cancer 136:E534–44
- Pandit V, Pai RS, Yadav V, et al. (2013). Pharmacokinetic and pharmacodynamic evaluation of floating microspheres of metformin hydrochloride. Drug Dev Ind Pharm 39:117–27
- Park SH, Gammon SR, Knippers JD, et al. (2002). Phosphorylation-activity relationships of AMPK and acetyl-CoA carboxylase in muscle. J Appl Physiol 92:2475–82
- Patel HK, Patel PR, Brahmbhatt TJ, et al. (2011). Sustained release microparticles: a review. Am J PharmTech Res 1:108–26
- Pavan Kumar BP, Sarath Chandiran IS, Bhavya B, et al. (2011). Microparticulate drug delivery system: a review. Indian J Pharm Sci Res 1:19–37
- Pernicova I, Korbonits M. (2014). Metformin-mode of action and clinical implications for diabetes and cancer. Nat Rev Endocrinol 10:143–56
- Pi-Sunyer FX. (2005). Weight loss in type 2 diabetic patients. Diabetes Care 28:1526–7
- Raghuwanshi AS, Raghuwanshi AS, Jain UK. (2010). Oral controlled release metformin hydrochloride ion exchange resinate beads. Int J Biomed Adv Res (IJBAR) 1:103–8
- Raparla R, Talasila EGKM. (2012). Design and evaluation of floating drug delivery systems of Metformin with natural gums as release retarding polymers. Int J Adv Pharm 1:22–38
- Ravi Kumar MN. (2000). Nano and microparticles as controlled drug delivery devices. J Pharm Pharm Sci 3:234–58
- Ren J, Zhou Y, Zhang G, et al. (2015). Role of age-related decrease of renal organic cation transporter 2 in the effect of atenolol on renal excretion of metformin in rats. Eur J Drug Metab Pharmacokinet 40:349-54
- Rizza RA. (2010). Pathogenesis of fasting and postprandial hyperglycemia in type 2 diabetes: implications for therapy. Diabetes 59:2697–707
- Sankhyan A, Pawar PK. (2013). Metformin loaded non-ionic surfactant vesicles: optimization of formulation, effect of process variables and characterization. Daru 21:7
- Satheesh Madhav NVS, Kala S. (2011). Review on microparticulate drug delivery system. Int J PharmTech Res 3:1242–54
- Scheen AJ. (2005). Drug interactions of clinical importance with antihyperglycaemic agents: an update. Drug Saf 28:601–31
- Shivhare UD, Darakh V, Mathur VB, et al. (2009). Preparation and evaluation of metformin hydrochloride microcapsules. Res J Pharm Tech (RJPT) 2:559–62
- Shugarts S, Benet LZ. (2009). The role of transporters in the pharmacokinetics of orally administered drugs. Pharm Res 26:2039–54
- Singh MN, Hemant KS, Ram M, et al. (2010). Microencapsulation: a promising technique for controlled drug delivery. Res Pharm Sci 5:65–77
- Snima KS, Jayakumar R, Lakshmanan VK. (2014). In vitro and in vivo biological evaluation of o-carboxymethyl chitosan encapsulated metformin nanoparticles for pancreatic cancer therapy. Pharm Res 31:3361–70
- Snima KS, Jayakumar R, Unnikrishnan AG, et al. (2012). O-carboxymethyl chitosan nanoparticles for metformin delivery to pancreatic cancer cells. Carbohydr Polym 89:1003–7
- Spritzer PM, Motta AB, Sir-Petermann T, et al. (2015). Novel strategies in the management of polycystic ovary syndrome. Minerva Endocrinol 40:195–212
- Su YW, Lin YH, Pai MH, et al. (2014). Association between phosphorylated AMP-activated protein kinase and acetyl-CoA carboxylase expression and outcome in patients with squamous cell carcinoma of the head and neck. PLoS One 9:e96183
- Sweetman S, ed. (2007). Martindale: the complete drug reference. London: Pharmaceutical Press (Electronic version)
- Thulé PM. (2012). Mechanisms of current therapies for diabetes mellitus type 2. Adv Physiol Educ 36:275–83
- Varde NK, Pack DW. (2004). Microspheres for controlled release drug delivery. Expert Opin Biol Ther 4:35–51
- Viollet B, Guigas B, Sanz Garcia N, et al. (2012). Cellular and molecular mechanisms of metformin: an overview. Clin Sci (Lond) 122:253–70
- Wahdan-Alaswad R, Fan Z, Edgerton SM, et al. (2013). Glucose promotes breast cancer aggression and reduces metformin efficacy. Cell Cycle 12:3759–69
- Wang LC, Di LQ, Liu R, et al. (2013). Characterizations and microsphere formulation of polysaccharide from the marine clam (Mactra veneriformis). Carbohydr Polym 92:106–13
- World Health Organization. (2015). Diabetes. Available from: http://www.who.int/mediacentre/factsheets/fs312/en/ [last accessed 11 Sep 2015]
- Zhang J, Shen C, Wang L, et al. (2014). Metformin inhibits epithelial-mesenchymal transition in prostate cancer cells: involvement of the tumor suppressor miR30a and its target gene SOX4. Biochem Biophys Res Commun 452:746–52
- Zhou G, Myers R, Li Y, et al. (2001). Role of AMP-activated protein kinase in mechanism of metformin action. J Clin Invest 108:1167–74
- Zhou M, Xia L, Wang J. (2007). Metformin transport by a newly cloned proton-stimulated organic cation transporter (plasma membrane monoamine transporter) expressed in human intestine. Drug Metab Dispos 35:1956–62