Abstract
Abstract: Alkaline phosphatase (ALP) was immobilized with cross-linking agents glutaraldehyde and cysteamine by forming a self-assembled monolayer on a screen printed gold electrode. ALP converts p-nitrophenyl phosphate to p-nitrophenol and phosphate. p-Nitrophenol loses H+ ion and turns into the negatively charged compound p-nitrophenolate at medium pH. As a result, the unstable product formed is measured chronoamperometrically at an application potential of + 0.95 V. The biosensor response depends linearly on p-nitrophenyl phosphate concentration between 0.05 – 0.6 mM with a response time of 40 seconds. Detection limit of the biosensor is 0.033 mM.
INTRODUCTION
Phosphatases (also called phosphomonoesterases) catalyze the hydrolysis of monoesters of orthophosphoric acid regardless of the kind of alcohol bound to a phosphate. They belong to non-specific enzymes that participate in photosynthesis, and other metabolic processes related to obtaining energy by organisms. Alkaline phosphatase (ALP), with activity optima at alkaline pH, is an enzyme whose activity is important in the milk industry to control pasteurization. It is widely employed in clinical diagnostics of various diseases. Alkaline phosphatase is widely used as an enzyme in immunoassays due to its high turnover number, broad substrate specificity, and the possibility of application to determining its activity by using various spectrophotometric and electrochemical methods [Citation1–4].
Alkaline phosphatase (E.C. 3.1.3.1) is a homodimeric enzyme that catalyzes the hydrolysis and transphosphorylation of a wide variety of phosphate monoesters. In recent years, phosphatases have found increasing application in design of enzymatic biosensors and immunosensors, where they are used as labels. It catalyses the hydrolysis of numerous substrates that contain a phosphate group. The choice of substrates for analytical applications depends (a) on the method of detection employed for monitoring the product of biocatalytic reaction and (b) on the purpose of analytical measurements. This may be (a) determination of the activity of ALP, (b) determination of various analytes based on inhibition of ALP activity, (c) immunoassay with ALP label, or (d) even the determination of inorganic orthophosphate [Citation2].
Investigations of the possibility of using different substrates are mostly carried out with dissolved ALP. Besides the commonly used p-nitrophenol phosphate, orthophosphate monoesters of phenol, α-naphthol, p-aminophenol, 4- methylumbelliferon, and 4-amino-1-naphthol have also been used to be a substrate of ALP enzyme [Citation5–9].
Interest in the properties of thin-film organic materials, especially self-assembled monolayers (SAMs), has grown considerably in recent years, primarily due to the ease of fabrication, characterization, and manipulation. The SAMs have been used in various areas such as molecular electronics, tribology, sensor fabrication, studies of electron transfer kinetics, and biomolecular investigations [Citation10–13].
Self-assembled monolayers (SAMs) on thin metal films have attracted a lot of attention in nanoscience and nanotechnology. They are easy to prepare and useful in studying interfacial phenomena, which is highly influenced by nanometer-scale topographies and composition. SAMs are formed by spontaneous adsorption of the molecular constituents from solution or gas phase due to its high affinity for a particular surface [Citation14,Citation15]. Self assembled monolayers (SAMs) of organosulfur compounds such as alkane thiols and disulfides on Au surface have received much attention in the past two decades due to their potential applications in the field of electroanalytical chemistry [Citation16]. The SAMs have electrochemical sensing applications and served as a platform for the fabrication of biosensors. Self-assembled monolayer (SAM) formation induced by the strong chemisorption between the substrate and head group of selected organic molecule provides one of the most elegant approaches towards making ultrathin organic films of controlled thickness [Citation16,Citation17].
One advantage of the self-assembling method over the other methodologies is that thickness of the monolayer can be easily controlled by choosing a suitable molecule. The existence of specific interactions between SAMs and the analyte of interest makes the self-assembling method more attractive in comparison to other modification methodologies. Good selectivity and high sensitivity can be achieved by the use of SAMs with suitable functional groups [Citation18–20].
In this paper a new biosensor, which was based on immobilization of alkaline phosphatase on a corundum ceramic-based screen printed gold electrode (1.0 mm), has been developed and adapted to p-nitrophenyl phosphate determination. In the biosensor construction, the enzyme was immobilized by using cross-linking agent glutaraldehyde and cysteamine by forming a self-assembled monolayer (SAM) on the electrode.
MATERIAL AND METHODS
Chemicals
Alkaline phosphatase (EC 3.1.3.1) from bovine intestinal mucosa, glutaraldehyde (25 %), cysteamine, p-nitrophenyl phosphate disodium salt hexahydrate, potassium chloride, magnesium chloride, KH2PO4, K2HPO4, glycine, and all the other chemicals were purchased from Sigma Chemical Co. (USA).
Electrochemical experiments were carried out in glycine buffer (50 mM; pH 10.5 containing 0.1 M KCl + 1 mM MgCl2).
Apparatus
Electrochemical measurements were performed using a PalmSens Instruments BV (Holland). Corundum ceramic-based screen printed gold electrode (1.0 mm) BVT Technologies (CZ) was used as a working electrode that is combined with the reference Ag/AgCl and the auxiliary AuPd (98/2%) electrode. In the experiments, Gibson P100 and P1000 automatic pipets (France), a yellow line magnetic stirrer (Germany), and a Nuve model thermostat (TR) were used. Ultra pure water used in the preparation of solutions was obtained from Mili-Q and Milipore RIOS-DI 3 UV (USA) water purification system.
Electrode Preparation
Corundum ceramic-based screen printed gold electrode was immersed in 100 mM cysteamine (Cys) solution (100 mM, in phosphate buffer; 50 mM, pH 7.0) to form the structure of a self assembled monolayer (SAM) during a 12-hour period. At the end of the period, the screen printed electrode was washed with ultra pure water and cross-linked with glutaraldehyde (5%) solution (in phosphate buffer; 50 mM, pH 7.0) for 30 min. At the end of the period, the electrode was washed again and immersed in alkaline phosphatase solution (32.5 Uml−1; with Tris-HCl buffer pH 7.0, 50 mM, contained MgCl2) for 22 hours. Thus, the immobilization of the enzyme was carried out. Finally, the electrode was washed with ultrapure water to remove unbonded enzyme molecules. shows the steps of the electrode preparation.
Chronoamperometric Measurements
Under alkaline conditions (pH > 10.0) ALP converts p-nitrophenyl phosphate (p-NPP) to p-nitrophenol and phosphate. p-nitrophenol loses H+ ion and turns into the negatively charged compound p-nitrophenolate at medium pH. This compound can irreversibly oxidize to p-nitro phenoxy cation at an anode resulting in a peak at + 0.95V [Citation21]. This product is measured chronoamperometrically at an application potential of +0.95 V [Citation22–25]. The anodic current values result from the oxidation of the enzymatically generated p-nitrophenol or p-nitrophenolate related to substrate concentration were recorded.
When the substrate was not in the reaction medium, the reaction did not occur and only background current was monitored at +0.95 V potential. After addition of substrate into the reaction medium, there is a current related to substrate concentration and the current was monitored as a function of time related to substrate concentration. Measurements were carried out in 0.1 M Glycine buffer (pH 10.5 containing 1 mM MgCl2, 0.1 M KCl) at 30°C.
RESULTS AND DISCUSSION
Cyclic Voltammograms Obtained by Screen-printed Electrodes
shows typical cyclic voltammogram obtained by the biosensor in the presence of 0.1M Glycine buffer and in the absence of substrate. Upon addition of 0.2 mM p-nitrophenyl phosphate (p-NPP) into the reaction cell a striking change in the voltammogram occurs (). According to the figure, in the presence of p-nitrophenyl phosphate an anodic peak current formed at between + 0.7 and +0.96 V potential range. This peak current resulted from a negatively charged compound, p-nitrophenolate, under alkaline conditions.
Figure 2. Cylic voltammograms obtained with the screen printed electrode: a) 0.1M Glycine buffer (pH 10.5 containing 1mM MgCl2 and 0.1M KCl) without p-nitrophenyl phosphate; b) with 0.2 mM p-nitrophenyl phosphate in 0.1 M glycine buffer (pH 10.5 containing 1mM MgCl2 and 0.1M KCl), T: 30°C. Scan rate is 50 mVs−1 and potentials were referred to Ag/AgCl reference electrode.

Optimization of Experimental Parameters
Influence of pH on the Biosensor Response. For most of the enzymes, optimum activity is achieved in a narrow pH interval. Outside this range, the enzymatic activity decreases due to changes in the enzyme’s structure and also affects the electrochemical response of the biosensor. The pH value of the electrolyte affects the three-dimensional structure of the enzyme. For the determination of the effect of pH value on the biosensor response different pH values were investigated. For this purpose 50 mM concentration of glycine buffers containing 1 mM MgCl2 and 0.1 M KCl (pH 8.5, 9, 9.5, 10, 10.5, and 11) were prepared and used in the experiments. The optimum pH value was obtained to be 10.5. From pH 8.5 up to 10.5 increases in the biosensor responses were observed. The biosensor response started to decrease when pH was above 10.5
The immobilization procedure did not affect the optimum pH value of alkaline phosphatase since both free and immobilized enzymes had the same optimum pH 10.5. shows the results obtained from the experiments.
Figure 3. The pH dependence on the peak current. 0.1 M glycine buffer containing 1 mM MgCl2 and 0.1 M KCl were used. Assay conditions: T: 30°C, applied potential: +950 mV, p-NPP concentration was 0.2mM.
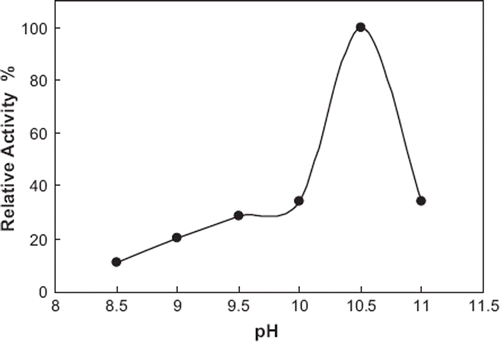
Effect of Temperature on the Biosensor Response. Since the enzyme activity is dependent on the temperature, the biosensor responses were measured at different temperatures ranging from 20 to 40°C. Increase in temperature caused higher signals until 30°C. However, at temperatures higher than 30°C a little decrease in the response was observed. Hence, 30°C was chosen to be the most suitable temperature value and used for all measurements.
Optimization of Bioactive Layer
To determine the effect of enzyme and glutaraldehyde amount, and SAM duration on the biosensor responses, different amounts of enzymes, glutaraldehyde concentrations, and different duration of SAM were carried out.
Duration of SAM Formation
The structure and properties of the SAMs on metal electrodes can be affected by the pretreatment processes of metal electrodes and the experimental conditions during the formation of SAMs, such as the immobilization technique, the concentration and composition of molecules or electrolyte solution, the temperature of self-assembly, etc.
For the investigation of the duration of SAM formation corundum ceramic-based screen printed gold electrodes were immersed in 100 mM cysteamine solution for 6, 12, 24, and 36 hours, respectively. The duration of SAM deposition was also varied because of the influence of the density of hydrocarbon chains in the SAM on the electrode. Results obtained from the experiments are shown in .
Figure 4. The effect of treatment time with cysteamine on biosensor response. (0.1 M glycine solutions containing 1 mM MgCl2 and 0.1 M KCl; pH 10.5, T: 30°C, applied potential: +950 mV). The electrode treatment with cysteamine for 6, 12, 24, and 36 hours and cross-linked with 5.0 % glutaraldehyde solutions. The enzyme amount was 32.5 unit/ml.
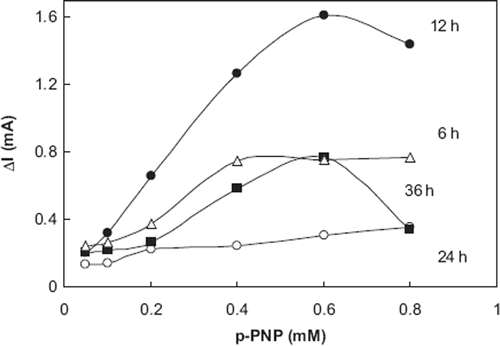
According to the results obtained from the experiments, the electrode that was immersed 12 h had highest biosensor responses for p-nitrophenyl phosphate. Whether the electrode had been immersed for 24 or 36 h, responses of the biosensor decreased dramatically as a result of deformations on SAM related to duration time. When the electrode was immersed for 6 h, linearity of calibration curve was deteriorated. From the results of all the experiments in the optimization of bioactive layer, it can be said that the most suitable biosensor responses correlated and also standard graphs were obtained with the 12 h duration of SAM.
Influence of Glutaraldehyde Concentration on the Enzymatic Activity. To determine the effect of glutaraldehyde (GA) concentration in the biosensor responses, cysteamine modified Au electrodes were cross-linked with different concentrations of glutaraldehyde (5.0%, 7.5%, and 10.0%). The results demonstrated that the biosensor response using t.5 and 10.0% glutaraldehyde was lower than that obtained with 5.0%. Although the biosensor responses obtained with 7.5 and 10.0% glutaraldehyde were nearly the same, the lowest biosensor responses were obtained by using 10.0% glutaraldehyde. From the experiments the best biosensor responses were obtained when 5.0 % glutaraldehyde was used. As a result, the most suitable glutaraldehyde concentration in the construction of the biosensor was chosen to be 5%.
Detection of the Effect of the Activity of the Enzyme on the Biosensor Responses. To determine the effect of alkaline phosphatase activity on the biosensor response, different enzyme amounts were used. For this purpose 16.25, 32.5, and 65 Uml−1 enzyme activity values were used in the biosensor preparation. Amperometric measurements were done in glycine buffer (pH 10.5) containing 1mM MgCl2 and 0.1 M KCl, at +0.95V.
From the results, higher biosensor responses and the most suitable calibration curve was obtained by the biosensor that contained 32.5 Uml−1 enzyme activity. When higher enzyme activity (65 Uml−1) was used in the biosensor construction, some deformations and diffusion barrier occurred in SAM surface. As a result of this effect, the biosensor responses decreased dramatically. In the case of 16.25 Uml−1 enzyme activity, biosensor responses were decreased and also linearity of calibration curve was swerved. This situation probably resulted from incapable bonding of enzyme molecules to the glutaraldehyde molecules.
Analytical Characteristics of the Biosensor
Linear Range for p-nitrophenyl Phosphate. Some amperometric measurements were taken to confirm responses of the biosensor for p-nitrophenyl phosphate. presents the results on the steady-state current at Cys-GA-ALP coated screen printed gold electrode in solutions containing different concentrations of p-nitrophenyl phosphate. The data in the experiments were acquired at a static potential (+0.95 V vs. Ag/AgCl).
Figure 5. Amperometric currents obtained for different p-NPP concentration. (a) 0.05 mM, (b) 0.1 mM, (c) 0.2 mM, (d) 0.4 mM, (e) 0.6 mM.
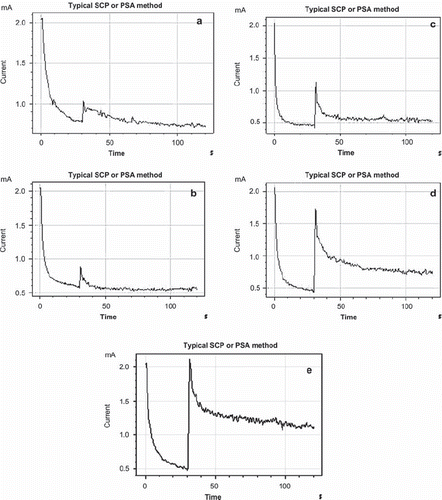
Responses of biosensor depend linearly on p-nitrophenyl phosphate concentration between 0.05–0.6 mM. Calibration curves obtained from the experiments are shown in .
Figure 6. The standard curve of the alkaline phosphatase biosensor (0.1 M glycine solutions containing 1 mM MgCl2 and 0.1 M KCl; pH 10.5, T: 30°C, applied potential: + 950 mV). The electrode was treated with cysteamine for 12 hours and cross-linked with 5.0 % glutaraldehyde solutions. The enzyme amount was 32.5 unit/ml.

The amperometric results indicated that when concentrations of p-nitrophenyl phosphate were increased, the corresponding biosensor responses also increased.
The enzymatic reaction rate is a linear function of the amount of the substrate at low concentrations. shows the influence of substrate concentration on the peak current. From 0.05 mM and 0.6 mM concentrations of p-nitrophenyl phosphate there was a linear relationship between substrate concentrations and the current peaks. At these concentrations, all the active sites of the enzyme are occupied by substrate molecules and the reaction rate is only dependent on the enzyme concentration.
Repeatability. In the repeatability experiments, 0.2 mM p-NPP standard was used. Eight injections (n = 8) were made with 0.2 mM p-NPP. The average value (x), the standard deviation (SD), and coefficient of variation (CV) were calculated to be 0.199 mM , ± 0.0117 mM, and 5.87 %, respectively. The limits of detection were calculated according to (3Sb/m) formula, where m is the slope of the calibration curve and Sb is the standard deviation (n = 8) of the response from 0.2 mM p-NPP. From the experiments detection limit was calculated to be 0.033 mM.
CONCLUSION
In the paper, an amperometric biosensor based on alkaline phosphatase has been described. The biosensor was prepared by using cysteamine-glutaraldehyde modified Au electrode. In optimization studies of the biosensor, some parameters, such as optimum pH, optimum temperature, effect of enzyme amount, and duration of SAM formation were investigated. We found that the best results were achieved at 30°C with glycine buffer (pH 10.5, 50 mM), 12 h SAM duration. Furthermore, the biosensor has a decent reproducibility for p-NPP.
Declaration of interest: The authors report no conflicts of interest. The authors alone are responsible for the content and writing of the paper.
REFERENCES
- Stec, B., Holtz, K.M., Kantrowitz, E.R. (2000). A Revised Mechanism for the Alkaline Phosphatase Reaction Involving Three Metal Ions. J. Mol. Biol. 299: 1303–1311.
- Szydłowska, D., Campas, M., JMarty, J.L., Trojanowicz, M. (2006). Catechol monophosphate as a new substrate for screen-printed amperometric biosensors with immobilized phosphatases. Sens. Act. B: Chemical 113: 787–796.
- Wilińska, A., Bryjak, J., Illeová, V., Polakovič, M. (2007). Kinetics of thermal inactivation of alkaline phosphatase in bovine and caprine milk and buffer. Int. Dairy J. 17: 579–586.
- Serra, B., Reviejo, A.J., Pingarrón, J.M. (2007). Application of electrochemical enzyme biosensors for food quality control. Comp. Anal. Chem. 49: 255–298.
- Wilson, M.S., Rauh, R.D. (2004). Hydroquinone diphospahte: an alkaline phosphatase substrate that does not produce electrode fouling in electrochemical immunoassays. Biosens. Bioelectron. 20: 276–283.
- Del Carlo, M., Lionti, I., Taccini, M., Cagnini, A., Mascini, M. (1997). Disposable screen-printed electrodes for the immunochemical detection of polychlorinated biphenyls. Anal. Chim. Acta 342: 189–197.
- Kronkvist, K., Lövgren, U., Svenson, J., Edholm, L.S., Johansson, G. (1997). Competitive flow injection enzyme immunoassay for steroids using a post-column reaction technique. J. Immun. Met. 200: 145–153.
- Thompson, R.Q., Porter, M., Stuver, C., Halsall, H.B., Heineman, W.R., Buckley, E., Smyth, M.R. (1993). Zeptomole detection limit for alkaline phosphatase using, 4-aminophenylphosphatase, amperometric detection, and an optimal buffer system. Anal. Chim. Acta 271: 223–229.
- Masson, M., Runarsson, O.V., Johannson, F., Aizawa, M. (2004). 4-Amino-l-naphthyl phosphatase as a substrate for the amperometric detection of alkaline phosphatase activity ands its application for immunoassay. Talanta. 64: 174–180.
- Shervedani, R.K., Hatefi-Mehrjardi, A., Babadi, M.K. (2007). Comparative electrochemical study of self-assembled monolayers of 2-mercaptobenzoxazole, 2-mercaptobenzothiazole, and 2-mercaptobenzimidazole formed on polycrystalline gold electrode. Electrochim. Acta 52: 7051–7060.
- Einati, H., Mottel, A., Inberg, A., Shacham, Y. (2009). Electrochemical studies of self-assembled monolayers using impedance spectroscopy. Electrochim. Acta. 54: 6063–6069.
- Shamsipur, M., Kazemi, S.H., Alizadeh, A., Mousavi, M.F., Workentin, M.S. (2007). Self-assembled monolayers of a hydroquinone-terminated alkanethiol onto gold surface. Interfacial electrochemistry and Michael-addition reaction with glutathione. J. Electroanal. Chem. 610: 218– 226.
- Da, C., Jinghong, L. (2006). Interfacial design and functionization on metal electrodes through self-assembled monolayers. Sur. Sci. Rep. 61: 445–463.
- Ang, X.F., Li, F.Y., Wei, J., Tan, W.L., Wong, C.C. (2008). A thermal and passivation study of self-assembled monolayers on thin gold films. Thin Sol. Films, 516: 5721–5724.
- Quist, F., Kakkar, A. (2007). Self-assembled monolayers: Influence of complementarity between chemisorbed and crystallizing molecules in polymorph selection. J. Coll. Int. Sci. 313: 378–382.
- Behera, S., Raj, C.R. (2007). Self-assembled monolayers of thio-substituted nucleobases on gold electrode for the electroanalysis of NADH, ethanol and uric acid. Sens Act. B: Chemical 128: 31–38.
- Chaki, N.K., Vijayamohanan, K. (2002). Self-assembled monolayers as a tunable platform for biosensor applications. Biosens. Bioelectron. 17: 1–12.
- Subramanian, S., Sampath, S. (2007). Enhanced stability of short- and long-chain diselenide self-assembled monolayers on gold probed by electrochemistry, spectroscopy, and microscopy. J. Coll. and Int. Sci. 312: 413–424.
- Arya, S.K., Solanki, P.R., Datta, M., Malhotra, B.D. (2009). Recent advances in self-assembled monolayers based biomolecular electronic devices. Biosens. Bioelectron. 24: 2810–2817.
- Shervedani, R.K., Bagherzadeh, M., Mozaffari, S.A. (2006). Determination of dopamine in the presence of high concentration of ascorbic acid by using gold cysteamine self-assembled monolayers as a nanosensor. Sens. Act. B: Chemical 115: 614–621.
- Zhu, X., Shi, S., Wei, J., Lv, F., Zhao, H., Kong, J., He, Q., Ni, J. (2007). Electrochemical oxidation characteristics of p-substituted phenols using a boron-doped diamond electrode Environ. Sci. Technol. 41: 6541–6546.
- Das, J., Jo, K., Lee, J.W., Yang, H. (2007). Electrochemical Immunosensor Using p-Aminophenol Redox Cycling by Hydrazine Combined with a Low Background Current. Anal. Chem. 79: 2790–2796.
- Bolado, P.F., Garcia, M.B.G., Garcia, A.C. (2006). Flow screen-printed amperometric detection of p-nitrophenol in alkaline phosphatase-based assays. Anal. Bioanal. Chem. 385: 1202–1208.
- Patil, S.J., Zajac, A., Zhukov, T., Bhansali, S. (2008). Ultrasensitive electrochemical detection of cytokeratin-7, using Au nanowires based biosensor Sens. Act. B: chemical 129: 859–865.
- Ionescu, R.E., Abu-Rabeah, K., Cosnier, S., Durrieu, C., Chovelon, J.M., Marks, R.S. (2006). Amperometric algal Chlorella vulgaris cell biosensors based on alginate and polypyrrole-alginate gels. Electroanal. 18: 1041–1051.