Abstract
Abstract: In this study, inkjet bio-printing has been used to produce miniaturized alginate microcapsules. A parametric study using subsequent Taguchi L18 (31 × 27) and L16 (45) designs was performed to elucidate the effect of inkjet parameters on microcapsule size. A 120-minute pilot run using the optimal waveform parameters and 0.5% alginate ink yielded a throughput of 1.8×106 microcapsules/hr, averaging 40 μm in diameter. Real-time stable jetting conditions were confirmed visually by the generation of a single droplet with a straight trajectory and non-fluctuating Ohnesorge numbers. The rate of stirring of the cross-linking CaCl2 solution determined scaffold vs. single vesicle formation.
INTRODUCTION
Bio-printing is used in many bioengineering applications, namely microencapsulation of cells and enzymes [Citation1] and nanodispensing in genomics [Citation2]. Depending on the desired print volume and precision, the driving force for the generation of the drop(s) falls into multiple categories. With the advances in stem cell technology the demand for reliable, high throughput production of smaller (<100 microns) artificial cells is increasing because of the following reasons: 1) diffusion of healing nutrients across the membrane is inversely proportional to the microcapsule radius (S/V) [Citation3, Citation4]; 2) the reduction in microcapsule size allows more choices of implantation sites [Citation5]; 3) encapsulating a single cell vs. multiple allows conducting single cell vs. population studies in an appropriate stem cell niche [Citation6]; 4) the amount of waste to be re-absorbed post-bio-membrane degradation can be reduced by 100% when scaling down from a diameter of 500μm to 100 μm for a 10-μm-thick membrane.
High-throughput production has been impeded by lack of methodology for miniaturized droplet generation. Previously, researchers have reported the fabrication of alginate microcapsules/scaffold and tubules of 50 µm diameter using inkjet technology [Citation7]. However, neither the rheological properties of the bio-ink, print-head rating, jetting parameters, and throughput nor the spreading ratios (Ri) have been reported. Without knowledge of the miniaturization parameters, results cannot be replicated nor can applications be refined amongst the research community. Reported miniaturization techniques for alginate-based microcapsules are electro-spraying and inkjet printing [Citation8, Citation7]. The inkjet method was chosen over electro-spraying because of commercial equipment availability and track record of reliability [Citation9].
Using this bio-printing method, high speed alginate liquid jets are generated by the contraction of piezo crystals when electrical energy is applied. The droplet generation capabilities are dictated by the rating of the print-head in terms of lower and upper limits of dynamic viscosity (μ) and surface tension (γ) of the jetted formulation (bio-ink). In the case of inkjet printing, unstable jetting is characterized by the generation of multiple streams and droplets, non-straight trajectories and satellites, and fluctuating dimensionless numbers. Dimensionless numbers such as the Reynolds number (Re), the Weber Number (We), and the Ohnesorge numbers are used in printing systems to adapt the bio-ink rheological properties to the flow geometry as well to optimize the spreading ratio (Ri), Ri being the ratio of the final capsule size (D) to the jetted droplet diameter (d).
The Ohnesorge (Oh) number given by equation (1) relates the viscous forces to inertial and surface tension forces in terms of the formulation properties such as (μ), density (ρ), (γ) and jetting parameters, resulting in a specific droplet size (d). In inkjet printing, neither long elastic tails nor inertially induced satellite drops are desirable; consequently, one may seek Oh ≤ 1 [Citation10]. In addition, fluctuating Ohnesorge numbers are a sign of process instability in terms of the jet formation mechanism.
MATERIALS AND METHODS
Materials
All chemicals used to make the microcapsules were purchased from Sigma Aldrich: low molecular weight sodium-alginate (A0682, 12-80 kDa), polylysine-hydrobromide (P81333, 20-30 kDa), and low molecular weight chitosan (44,886-9, 75% deacetylated, 3.8-6 kDa). The piezo jetting devices serial numbers MJ-B10-10-02, MJ-B10-44-02 and MJ-B10-49-02 were purchased from Microfab Technologies. E. coli DH5 (ATCC 53868) was procured from American Type Culture Collection.
Methods
The experiments and physical set-ups were designed to meet the following original research objectives: (a) rheological characterization of the non-Newtonian jetting formulation for dimensional analysis; (b) artificial cell miniaturization by robust modeling prediction of jetted droplet sizes using Taguchi Designs; and (c) morphology study.
Experimental Set-up. Miniaturized microcapsules were fabricated using Microfab's Jetlab System presented in and . The apparatus consists of a CCD camera (30 fps), a control unit, a print-head, a triggering unit, a fluid delivery unit, and a PC equipped with proprietary software (MicroFab JetServer) to tune the bio-ink formulation to the waveform parameters [rise (µsec), dwell Time (µsec), fall (µsec), echo (µsec), final rise (µsec),frequency (Hz), voltage (V), drops/trigger]. The print-head has an aperture of 60 μm. After inputting the jetting variable settings, droplet generation begins with the triggering box sending electrical signals to the ink-jet control unit and to the CCD camera control PC, simultaneously. The ink-jet engine fires through the inkjet head into the 10% (w/v) CaCl2 cross-linking solution. The CCD camera captures a picture of the droplet exiting the print-head. By controlling the delay time of the camera through software, the picture of the droplet can be taken at different points prior to the impacting process. The imaging software has a motion analysis feature that captures droplet size and velocity.
Figure 1. (a) Inkjet printing equipment and software interface. Shown on the left is an image of the sodium alginate jet. Captured on the right is an image of the waveform, waveform parameters, and the results of the motion analysis software (below). (b) The actual physical SJSU set-up.
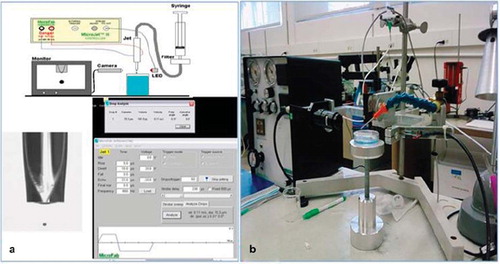
Apart from the waveform parameters, the solution height is defined as the distance between the printhead tip and the cross-linking solution. Results of prior screening experiments indicated that a range of 1-4 mm is ideal for a spreading ratio (Ri) of 0.5.
Rheological Characterization of the Non-Newtonian Jetting Formulation for Dimensional Analysis. Bio-ink formulation is limited by the piezo print-head rating in terms of surface tension and viscosity (μ < 40 cP and γ < 0.07 N/m).
Bio-ink surface tension was characterized by the sessile drop method using a calibrated CCD camera. The software CamExpert (SAPERA V.6.30.00.0811) is used to capture droplet images. The captured meniscus heights are analyzed using ImageJ (V1.42). An average of six measurements were conducted to calculate the surface tension of the bio-ink using Young's equation relating (γ) to the contact angle [Citation11]. For viscosity measurements, formulations were characterized using Canon-Ubbelohde Semi-Micro capillary viscometers (sizes: 75, 100), where kinematic viscosity (η) is converted to dynamic viscosity (μ) by multiplying the (η) by solution density.
Dimensional analysis was facilitated by real-time monitoring of the droplet size and velocity by the motion analysis software depicted by and . The vision system was calibrated by finding the CCD camera vertical focal distance; the focal distance was determined in turn by finding the length per pixel ratio using ImageJ (V1.42). (Re) and (Oh) numbers were calculated by using bio-ink density, surface tension, and viscosity, as well as jetted droplet size and velocity.
Artificial Cell Miniaturization by Robust Modeling Prediction of Jetted Droplet Sizes using Taguchi Designs. The software Minitab (v16) was used for data processing and analysis throughout the experimental design phase. Both optimizations have been conducted using the same print-head (serial #MJB10-10-02).
Screening Design 1: Model Bio-ink. An L18 (31 3 27) Taguchi design comprised of 8 parameters [final rise (µsec), dwell time (µsec), fall (µsec), echo (µsec), frequency (Hz), voltage (V), drops/trigger, and solution height (mm)] was conducted on a Newtonian bio-ink comprised of 10% PEG 4000 dissolved in saline (μ = 2.7 cP, γ = 0.053 N/m). The binary response was a {jet, no jet/0,1} response and the type of optimization was Nominal the Best. For each experimental run the solution was allowed to jet for 5 minutes and vision system analysis was collected every minute.
The main effects on droplet size and S/N ratios were used in turn as variables for the next design L16 (45) Taguchi matrix.
Design 2: Non-Newtonian Alginate Bio-ink. A L16 (45) Taguchi matrix comprised of 5 parameters [voltage (V), frequency (Hz), drops/trigger, dwell time (µsec), and solution height (mm)] was conducted. The responses were droplet size and number of droplets.
For each experimental run the solution was allowed to jet for 5 minutes and vision system analysis was collected every minute.
For both responses the type of optimization was Nominal the Best. Based on prior one factor at a time experiments, the target for drop size and number of drops were 85 μm and 1.0, respectively.
For the confirmation run, random sampling of the vision system output in terms of droplet size and droplet number was conducted every 24 minutes throughout the 120-minute run.
The velocity and droplet size were recorded for each run in order to monitor the change in dimensionless numbers.
A backpressure of 21.2 psi was maintained throughout the experiments. The other waveforms parameters were set at the following nominal values for all L16 runs: rise = fall = 5 µsec; final rise = 3 µsec; echo = 10 µsec.
Morphology Study. An air jet stream was designed to stir the 10% (w/v) CaCl2 cross-linking solution at various rates ranging from 1-14 L.min−1. The objective was to determine the effect of stirring on alginate structure formation upon impact/contact with the CaCl2 solution.
RESULTS
The optimal bio-ink was determined to be 0.5% alginate solution dissolved in saline characterized by µ = 5 ± 0.2 cP and γ = 0.041 ± 0.006 N/m. This formulation was used throughout the L16 design. For the sterilized alginate used to immobilize bacteria in order to give the captured pictures a scale perspective, the initial alginate concentration was 1%.
As mentioned earlier, formulation properties were constrained by the Microfab Technologies inkjet (60 μm orifice) print-head rating of 40 cP and 0.07 N/m, and the non-Newtonian nature of the Alginate bio-ink. At the nominal 1.5% CaCl2 concentration used for atomization [Citation12], the jetted droplet dissociated upon impact due to the 100-fold reduction in viscosity. To overcome this challenge, the concentration of CaCl2 was increased from the nominal 1.5% to 10%.
As indicated by the results of the first screening design (Taguchi L18) presented in , voltage (V), frequency (Hz), drops/trigger, dwell time(µsec), and solution height (mm) govern the feasibility of jetting. These findings were used to select the factors for the subsequent (L16) matrix (). Main effect plots for the signal to noise (S/N) ratio and average responses for the L16 droplet size optimization are presented in and , respectively. The main effects controlling droplet size and hence capsule size are voltage, frequency, and solution height. A similar analysis was conducted for the prediction of droplet number and the same pareto of effects was obtained. The recommended operating conditions for obtaining a target droplet size of 85 μm (<100 μm) and single droplet formation corresponding to a capsule sizes of 40 μm ± 10 μm (Ri = 0.5) are summarized in .
Table 1. Summary of Taguchi L18 (31 × 27) results
Table 2. Summary of Taguchi L16 (45) results
Table 3. Summary of droplet size optimization results
No satellites were observed throughout a pilot 120-minute confirmation run yielding a throughput of 1.8×106/hr calculated based on a jetting frequency of 500 Hz. The jetting throughout this pilot run was characterized by a laminar regime (Re << 2000). An average Oh of 0.085 with a coefficient of variability (CV) of 0.93% were calculated for the pilot confirmation run. The average Ohnesorge number being close to unity [Citation10] and a %CV of less than 1% indicate reliable jetting conditions.
In the absence of air flow, the alginate droplets merged upon impact and formed amorphous tubules or scaffolds in the cross-linking solution as shown in . The formation of these structures is irreversible. An air flow rate of 2/L.min-1 is sufficient to prevent vesicle fusion and allows for distinct capsule formation (). As illustrated in , E. coli DH5 bacteria pre-mixed with the jetted alginate has been immobilized in the miniaturized artificial cells [Citation14]. Bacterium with an average size of 1μm has been chosen to add some perspective to the miniaturization process. As stated above, the miniaturized cell population was characterized by a diameter of 40 μm ± 10 μm with an approximate membrane thickness of 1.5 μm.
Figure 3. (a) Scaffold formation with no stirring of CaCl2 solution; (b) distinct vesicle formation at an air flow rate of 2 L/min−1 for stirring the cross-linking solution. Shown in highlighted circles are microencapsulated E. coli-DH5 [Citation14].
![Figure 3. (a) Scaffold formation with no stirring of CaCl2 solution; (b) distinct vesicle formation at an air flow rate of 2 L/min−1 for stirring the cross-linking solution. Shown in highlighted circles are microencapsulated E. coli-DH5 [Citation14].](/cms/asset/4e6978da-3670-4f38-b04b-587d69f6c5c9/ianb19_a_574637_f0003_b.jpg)
If not washed with saline and adsorbed with a polycation such as polylysine or chitosan immediately after fabrication, the miniaturized capsules fused irreversibly.
DISCUSSION
Membrane permeability has not been tested as a result of the increase in the CaCl2 concentration. It has been reported that cross-linking decreases the transition temperature of the alginate membranes and thus the permeability is independent of the guluronic acid content [Citation13].
Each piezo print-head supplied by Microfab Technologies is received with a different set of recommended operating conditions based on the standard 70IPA/30H2O% (v/v) used for testing. Due to the manufacturing process, the “squeeze volume” or volume of bio-ink upon jetting differs from print-head to print-head. Two other piezo jetting devices (MJ-B10-44-02 and MJ-B10-49-02) have been screened for capsule miniaturization. Although preliminary optimization results lead to a different set of operating conditions, the pareto of the main effects shows the same trend, indicating that the methodology described in this paper can be used to tune piezo devices to jet alginate solutions reliably.
Inkjet printing of alginate beads has been pioneered by Nakamura et al., but thus far none of the waveform parameters nor the rheological properties have been published [Citation7,Citation15].
It is not clear why fusion of the miniaturized capsules is irreversible if not coated immediately to create an electrostatic barrier. One hypothesis is that the 100-fold decrease in dynamic viscosity when going from the standard atomization technique to the inkjet method reduces the compressive strength of the membrane and renders the structures more compliant and susceptible to aggregation.
In a recent study, leaky/non-spherical alginate artificial cells were made from 0.5-1 % alginate solution using inkjet printing [Citation16]. The shape of the formed microcapsules was influenced by increasing the viscosity of the receiving CaCl2 (maximum 2% (w/w)) solution using glycerol and varied from elongated to spherical to flattened with average spreading ratios ranging from 0.7 to 1.2. The main factor influencing capsule size was identified to be the voltage applied. Droplet stability, dimensional analysis, and the trends for the other waveform parameters have not been disseminated. The disadvantage of using glycerol is the decrease in membrane strength as measured by the decrease in Young's modulus measured by researchers, who have used glycerol as a plasticizer for alginate films [Citation17].
CONCLUSION
In this study, using robust experimental techniques, 40 μm (±10 μm) and 1.5 μm thick spherical miniaturized microcapsules under known waveform parameters have been fabricated. In addition, dimensional analysis has been conducted in order to monitor process stability and characterize the jetting conditions. Next efforts will encompass two-fold permeability studies verifying the impact of the higher surface to volume ratios as a result of miniaturization, as well as the effect of the enhanced cross-linking at higher CaCl2 on the molecular weight cut-off of the membrane.
Declaration of interest: The authors report no conflicts of interest. The authors alone are responsible for the content and writing of the paper.
REFERENCES
- Chang, T.M.S. (2007). Artificial Cells: Biotechnology, Nanomedicine, Regenerative Medicine, Blood Substitutes, Bioencapsulation, Cell/Stem Cell Therapy. London: Imperial College Press, London. [online] Available at: www.artcell.mcgill.ca for free access or download. Accessed on 2/1/2011.
- Abba, M.C., Hu, Y., Sun, H., Drake J.A., Gaddis, S., Baggerly, K., Sahin, A., Aldaz, C.M. (2005). Gene expression signature of estrogen receptor a status in breast cancer. BMC Genomics. [online] Available at: http://www.biomedcentral.com/1471-2164/6/37. Accessed on 2/1/2011.
- Uludag, H., De Vos, P., Tresco P. A. (2000). Technology of mammalian cell encapsulation. Adv. Drug Delivery Rev. 42(1–2): 29–64.
- Desai, L., Perkins, J., Harrison, B.S., Sankar, J. (2010). Understanding release kinetics of biopolymer drug delivery microcapsules for biomedical applications. Materials Science and Engineering B. B168: 127–131.
- Wilson, J.T., Chaikof, E.L. (2008). Challenges and emerging technologies in the immunoisolation of cells and tissues. Adv. Drug Delivery Rev. 60(2): 124–145.
- Karoubi, G., Ormiston, M. L., Stewart, D. J., Courtman, D. W. (2009). Single-cell hydrogel encapsulation for enhanced survival of human marrow stromal cells. Biomaterials, 30(29): 5445–5455.
- Henmi, C., Nakamura, M., Nishiyama, Y., Yamaguchi, K., Mochizuki, S., Takiura, K., Nakagawa, H. (2008). New approaches for tissue engineering: three dimensional cell patterning using inkjet technology. Inflammation and Regeneration, 28(1): 36–40.
- Wujie, Z., Xiaoming, H. (2009). Encapsulation of living cells in small (∼100 m) alginate microcapsules by electrostatic spraying: a parametric study. J. Biomech. Eng., 131(7): 074515-(1–6).
- Dufva, M. (2005). Fabrication of high quality microarrays. Biomol. Eng., 22(5–6): 173–184.
- McKinley, H. G. (2005). Dimensionless groups for understanding free surface flows of complex fluids. SOR Rheology Bulletin. [online] Available at: www.rheology.org/sor/publications/Rheology_B/RB2005Jul-a.pdf. Accessed 2/1/2011.
- Fournier, R.L. (1998). Basic Transport Phenomena in Biomedical Engineering, 2nd. Philadelphia: Taylor & Francis.
- Prakash, S., Chang, T.M.S. (2004). Preparation and in vitro analysis of microencapsulated genetically engineered E. coli DH5 cells for urea and ammonia removal. Biotech. and Bioeng., 46(6): 621–626.
- Russo, R., Malinconico, M., Santagata, G. (2007). Effect of cross-linking calcium ions on the physical properties of alginate films. Biomacromolecules, 8: 3193–3197.
- Shan, Y., Wong, J., Deol, S., Mobed-Miremadi, M., Selvaduray, G. (2011). High throughput encapsulation of E. Coli DH5 using inkjet bio-printing. Proceedings of the 23rd CSU Annual Biotechnology Symposium. [online] Available at: http://csuperb.org/oars/abstracts.php?p = author&o = 14. Accessed 2/1/2011.
- Nakamura, M., Nishiyama, Y., Henmi, C. (2008). 3D micro-fabrication by inkjet biofabrication for 3D tissue engineering. Micro-NanoMechatronics and Human Science. 451–456.
- Dohnal, J., Štěpánek, F. (2010). Inkjet fabrication and characterization of calcium alginate microcapsules. Powder Technology. 200: 254–259.
- Avella, M., Di Pace, E., Immirzi, B., Impallomeni, G., Malinconico, M., Santagata, G.. (2007). Carbohydrate Polymers. 69(3): 503–511.