Abstract
A novel highly sensitive electrochemical carboxymethylcellulose-gelatin-superoxide dismutase biosensor was used for the determination of superoxide radicals enhancement in tomato plants exposed to salinity, drought, cold and heavy metal stress. The variations in superoxide radicals depending on abiotic stress was determined using biosensor. The superoxide radical production with regard to control rapidly was increased in tomato plants exposed to salinity, drought, cold and heavy metal stress. The superoxide radical enhancement in tomato plants exposed to salinity, drought, cold and heavy metal stress was successfully determined using carboxymethylcellulose-gelatin-superoxide dismutase biosensor.
Introduction
Plants are exposed to a wide array of environmental stress factors such as drought, heat, cold, ultraviolent light or pathogen attacks etc (Mc Kersie and Leshem Citation1994, Paliyath et al. Citation1997). These factors affect many genetic, physiological and biochemical responses in plants For example, changes in membrane composition, closure of stoma, photosynthetic efficiency, accumulation of sugar or other compounds, production of small-molecules and free-radicals and activities of antioxidant enzymes and their gene expressions (Beck et al. Citation2007, Mahajan et al. 2005, Tian et al. Citation2011, Bowler et al. Citation1992, Foye et al. Citation1994).
When stress conditions are perceived by plants, three kind of stress signaling can occur. These are: (a) osmatic stress signaling to reestablishment of cellular homeostasis, (b) detoxification signaling to control or repair stress damage, (c) and signaling to coordinate cell expansion to levels appropriate for the particular stress condition (Zhu Citation2001).
Abscisic acid (ABA), phytohormone, plays an important role in enhancing tolerance against water, salt and cold stress. It modulates many significant development processes of plant; such as inhibition of germination, maintenance of seed dormancy, regulation of growth and stomatal closure (Finkelstein et al. 2002, Parent et al. Citation2009, Raghavendra et al. Citation2010). In consequence of stomatal closure and limited CO2 availability, chloroplasts mitochondria and peroxisomes generate reactive oxygen species (ROS) such as O2‐, H2O2, 1O2, HO2‐, OH, ROOH, ROO and RO which tend to act as detoxification signals at cellular level (Smirnoff Citation1993, Gratao et al. Citation2005, Mittler Citation2002).
Under normal growth condition, production of ROS is limited and there is equilibrium between production and scavenging of ROS. Many stress condition such as drought, desiccation, salt stress, chilling, heat shock, heavy metals, ultraviolent radiation, air pollutions etc. disturb the homeostasis and enhance ROS production (Bowler et al. Citation1992, Allen Citation1995, Dat et al. Citation2000). Enhanced production of ROS is critical for plants because overaccumulation of them can result cell death in plants (Asada and Takahashi Citation1987, Hammond- Kosack and Jones 1996). For scavenging of ROS, plants possess two types of antioxidant mechanism namely nonenzymatic antioxidants and enzymatic antioxidant systems. Glutathione (GSH), proline, carotenoids, tacoppherol etc. are called nonenzymatic antioxidants and monodehydroascorbate reductase (MDHAR), dehydro ascorbate reductase (DHAR), glutathione reductase (GR), ascorbate peroxidase (APX), catalase (CAT) and superoxide reductase (SOD) also called enzymatic antioxidants (Verma et al. Citation2003, Verma and Dubey. Citation2003, DalCorso et al. Citation2008).
SODs have been distinguished into different classes depending on the metal (manganese (Mn), iron (Fe), copper (Cu) and zinc (Zn)) at the active center. All have different localization in plants. For instance; Cu/Zn SODs are generally found in the cytosol or chloroplasts of eukaryotic cells, whereas MnSODs are found in the mitochondria, chloroplasts and peroxisomes. The dimeric FeSODs are found in chloroplasts. SODs play a significant role in detoxifications of toxic effects of oxidative stress by scavenging superoxide radicals, providing their conversion into oxygen and hydrogen peroxide. (Van Camp et al. Citation1994, Fridovich Citation1995, Salin and Bridges Citation1980, Del Río et al. Citation1983, Droillard and Paulin Citation1990).
Superoxide radicals (O2•−), the primary species of the ROS, produced in biological systems, play a central role in physiological processes (Auchere and Rusnak Citation2002, Dean et al. Citation1997, Halliwell and Gutteridge Citation1984). Superoxide radicals are generated at a rate that is matched by the capacity of tissue to catabolize them under normal metabolic conditions (Van Lente Citation1993). When its level exceeds the body's natural ability to deal with the potentially cytotoxic species, various pathological situations such as cancer, stroke, neurodegeneration and aging may result (Halliwell and Gutteridge Citation1986, Mannino et al. Citation1999, Pinzino et al. Citation1999, Hensley and Floyd Citation2002, Youdim and Joseph 2011). In plants, O2•− is commonly generated in illuminated chloroplasts by the occasional transfer of an electron from an excited Chl molecule or Photosystem I components under conditions of high NADPH/NADP ratios to molecular O2 (Scandalios Citation1993). In most aerobic organisms, different environmental complications, like hyperoxia, pathogens, ozone, temperature fluctuations, and other stresses are known to stimulate O2•− formation (Scandalios Citation1993). Therefore, in order to understand the role of O2•− in pathology and physiology and the relationship between O2•− and environmental stresses, the quantitative determination of O2•− in a variety of in vitro and in vivo models is essential. A major challenge of the quantification of superoxide radical in biological models is of its short duration due to its spontaneous dismutation to oxygen and hydrogen peroxide and low concentration (Fridovich Citation1972, Beissenhirtz et al. Citation2006). Determination of free radicals is usually performed by ESR (Carey and Sundberg Citation1984), chemiluminescence (Prasad et al. Citation1989) or certain semiquantitative colorimetric tests (Halliwell and Gutteridge Citation1981, Fridovich Citation1970). These indirect methods are ex situ detection techniques with low sensitivity and poor selectivity. However, among the analytical systems, electrochemical techniques have proven to be powerful tools with the advantage of simplicity, portability, cost, fast response, high selectivity and real-time measurements (Tammeveski et al. Citation1998a, Land and Swallow Citation1971, Rigo et al. Citation1992, Tanaka and Muto Citation1992, Moscone and Mascini Citation1988, McNeil et al. Citation1989, Oshaka et al. Citation1995, Tammeveski et al. Citation1998b).
Superoxide dismutases (SODs) which protect the organism against the toxic effects are ubiquitous metalloenzymes in oxygen-tolerant organisms and catalyze the dismutation of O2•− to O2 and H2O2 via a cyclic oxidation–reduction electron-transfer mechanism (Rotilio et al. Citation1972, Klug et al. Citation1972, Lawrence and Sawyer Citation1979). SOD, a selective scavenger of O2•−, has been the best approach for the electrochemical detection of O2•− compared to cyt c (Cooper et al. 1993, Lisdat et al. Citation1999). Therefore, SOD, a specific enzyme for O2•− dismutation, offers great potential for the sensitive and selective quantification of O2•− in electrochemical biosensors. Mostly, copper, zinc–SOD-immobilized electrodes have paved an elegant way to detect O2•−.
The development of a highly sensitive superoxide dismutase biosensor for the simultaneous determination of superoxide radicals by immobilization of SOD within carboxymethylcelulose-gelatin on a Pt electrode surface was performed (Kocabay et al. Citation2012). The parameters affecting the performance of the biosensor were investigated in detail. The response of the CMC–G–SOD biosensor was proportional to O2•− concentration and the detection limit was 1.25 × 10‐3 mM with a correlation coefficient of 0.9994 at a signal-to-noise ratio of 3. The response time of the developed biosensor was 2 seconds (Kocabay et al. Citation2012). In this study, the applications of developed CMC–G–SOD biosensor to agriculture were studied. For this purpose, tomato plants were exposed to heavy metal, cold, salinity and drought stress to examine the O2•− enhancement. The effect of heavy metal, cold, salinity and drought stress in tomato plants on O2•− enhancement was investigated using developed CMC–G–SOD biosensor.
Materials and methods
Materials
SOD (EC 1.15.1.1, 4733 U mg − 1, from bovine erythrocytes), xanthine oxidase (XOD; EC 1.1.3.22, 0.3 U mg −1, from milk), xanthine (2,6-dihydroxypurine) sodium salt, gelatin, carboymethylcelulose and chemicals used for preparation of buffers and cross-linking agents were purchased from Sigma (St Louis, MO). The current was measured with a Gamry Framework Version 5.50 Amperometry. Aspirin (500 mg acetylsalicylic acid per tablet) and aspirin containing vitamin C (400 mg acetylsalicylic acid and 240 mg ascorbic acid per tablet) were purchased from a pharmacy.
Electrolysis cell and electrodes
A three-electrode electrochemical cell comprising working, counter and reference electrodes was used for all experiments. The working (area 0.5 cm2) and counter electrodes were platinum; their surfaces were polished using 0.05 μm alumina slurry. The reference electrode was Ag/AgCl, introduced into the cell with a Luggin capillary.
Preparation of SOD biosensor
Polymer blends (CMC and gelatin) were prepared to obtain a final weight of 3,75 mg polymer in final solution by dissolving them in phosphate buffer (0.05 M, pH 7.4). SOD (4733 U, 1 mg) and glutaraldehyde (0.005 M) were added to the support system solutions to obtain a final volume of 50 μL at 32°C. The platinum foil electrode was covered with a total of 50 μL immobilization gel, 25. μL on each surface. The CMC–G–SOD electrodes were left at room temperature for 48 hour. Enzyme leakage tests were performed for all enzyme electrodes by washing with phosphate buffer (0.05 M, pH 7.4), three times, for 1 hour each, at 25°C. These tests were performed for all CMC–G–SOD biosensors obtained (Kocabay et al. Citation2012).
Principle of method
The biosensor used to determine the O2•− was obtained by coupling an amperometric electrode for hydrogen peroxide with the SOD enzyme immobilized on CMC–G support system (Kocabay et al. Citation2012).
The capacity of the SOD biosensor was determined as follows: the O2•− is produced in aqueous solution by oxidation of xanthine to uric acid in the presence of xanthine oxidase:
SOD immobilized on the Pt electrode catalyzes the dismutation reaction of the O2•− with release of oxygen and hydrogen peroxide according to equation
The H2O2 can be detected at the electrode surface in accordance with equation
The current generated by oxidation of hydrogen peroxide at the working electrode held at 650 mV relative to the Ag/AgCl electrode is proportional to the concentration of O2•− in solution. The reaction scheme on the electrode surface is as follows:
Measurement of procedure
All measurements were performed in a standard three-electrode thermostated Pyrex cell containing 15 mL 0.05 M phosphate buffer, pH 7.4, at 25°C. The working electrode was placed in the cell and allowed to stabilize with a constant stirring rate of 1,000 rpm.
The biosensor response was used to examine the O2•− enhancement in heavy metal, cold, salinity and drought stress-treated tomato plants. The stress-treated tomato plants (totally leaves, roots and stems) (0.5 g) were homogenized in distilled water (3 mL) using a Bandalin homogenizer. The biosensor was left to stabilize in a cell containing phosphate buffer (0.05 M, pH 7.4) with magnetic stirring, until the response became constant. A solution of homogenized stress-treated tomato plants (500 μL) was added and the biosensor response was recorded. The stress-treated tomato plants were stored at −20°C before use. All experiments were performed five times in this work.
Growth condition
Tomato (Lycopersicum esculentum L. ‘Falcon’) seeds were germinated and grown hydroponically in pots containing 0.2 L of modified 1/10 Hoagland's solution. Hoagland solution includes macronutrients (K2SO4, KH2PO4, MgSO4.7H2O, Ca (NO3)2. 4H2O and KCl) and micronutrients (H3BO3, MnSO4, CuSO4.5H2O, NH4Mo, ZnSO4.7H2O) with final concentrations of 2 mM Ca, 10-6 M Mn, 4 mM NO3, 2.10-7 M Cu, 1 mM Mg, 10-8 M NH4, 2 mM K, 10-6 M Zn, 0.2 mM P, 10-4 M Fe ve10-6 M B. Six plants were grown in each pot in a controlled environmental growth chamber with light of 250 mmol m–2 s–1 photosynthetic photon flux at 25°C, 70% relative humidity. Twenty-five-days-old plants grown in controlled media were used for stress treatments.
Stress treatment
Salinity and drought treatment
Twenty-five-days-old plants grown in controlled media were used for stress treatments. Treatment of the plants with salt was imposed by the addition of NaCl to the nutrient solution to a final concentration of 100 and 150 mM. Osmotic pressures of the salt solutions were determined by Vapor Pressure Osmometer 5520. The average osmotic pressure of 100 mM NaCl solution was estimated as 190 mmol/kg while 150 mM NaCl solution was found approximately as 290 mmol/kg. For the drought treatment (Verslues et al. 2006), PEG was added to the nutrient solution until the osmolality of the solution, as measured using a vapor pressure osmometer, was the same as that of the salt solutions. In this way, an iso-osmotic level of stress was applied to both the salt and PEG-treated plants, with the goal of matching the stem water potentials of the plants exposed to these treatments. These applications were named as 100 and 150 mM drought treatment. Six plants in a single pot were harvested at 0, 3, 6, 12, 24 and 48 hours after the plants were exposed to these treatments.
Cold treatment
For application of cold stress, plants were exposed to 4°C and samples were collected in different time periods (control, 2 days, 4 days, 6 days, 8 days and 10 days).
Heavy metal treatment
Twenty-five-days-old plants grown in controlled media were used for stress treatments. For application of heavy metal stress Cd2+ , Pb2+ , Cu2+ , Mn1+ were added to the hydroponic solution for 24 hours at concentrations of 0 (control), 80, 160, 320, 640 and 1280 mM.
Results and discussion
The development of highly sensitive SOD biosensor for the simultaneous determination of superoxide radicals by immobilization of superoxide dismutase within CMC–G on a Pt electrode surface was performed. The parameters affecting the performance of the biosensor were investigated in detail (Kocabay et al. Citation2012). The response of the CMC–G–SOD biosensor was proportional to O2•− concentration and the detection limit was 1.25 × 10‐3 mM with a correlation coefficient of 0.9994 at a signal-to-noise ratio of 3. The response time of the developed biosensor was 2 seconds (Kocabay et al. Citation2012). In this study, the application of developed CMC–G–SOD biosensor to the agriculture was studied. For this purpose, tomato plants were exposed to heavy metal, cold, salinity and drought stress to examine the O2•− enhancement. The effect of heavy metal, cold, salinity and drought stress in tomato plants on O2•− enhancement was investigated using the developed CMC–G–SOD biosensor.
Amperometric response of the G–SOD biosensor to O2•−
The amperometric response of the CMC–G–SOD biosensor resulted from superoxide anion was investigated in the stirred buffer solution. An efficient and simple method was utilized for generation of O2•− by oxidation of xanthine to uric acid in the presence of xanthine oxidase at an applied potential of 650 mV. The measurement solution should be operated at a constant and high stirring speed (1,000 rpm) to acquire reproducible results. A peak-like response was observed, which is in agreement with a report by other worker (Lisdat et al. Citation1999, Di et al. Citation2004, Fujita et al. Citation2009, Di et al. Citation2007, Wang et al. Citation2009). A calibration plot as a function of increasing xanthine concentration for the CMC–G–SOD biosensor was obtained previously by ourself (Kocabay et al. Citation2012) (). The amperometric response of the CMC–G–SOD biosensor increases with ascending xanthine concentration.
Salinity and drought treatment
The effect of drought stress in tomato plants on O2•− enhancement was investigated using developed CMC–G–SOD biosensor. The measurements were performed for a period of 48 hours. As can be seen from , with regard to control, the O2•− production rapidly increased for a 12 hour-period of both 100 and 150 mM drought-treated tomato plants. After this time period, profiles of O2•− production were differentiated between 100 and 150 mM drought-treated samples. O2•− production continued to increase and reached maximum level after 48 hours in 100 mM drought-treated tomato plants. On the other hand, 150 mM drought-treated tomato plants reached maximum O2•− production after 24 hours and then decreased, while O2•− enhancement was obtained by CMC–G–SOD biosensor for 100 mM drought-treated tomato plants.
Also, the O2•− productions were studied with the CMC–G–SOD biosensor for 100 and 150 mM salt-treated tomato plants (). The O2•− production significantly increased up to 9 days for 100 mM and 12 days for 150 mM salt-treated tomato plants. After this time period, decrease orientations were monitored in the O2•− productions. Profile of decrease was similar for all salt treatment but minimum O2•− level was recorded in 100 mM salt-treated tomato plants for 48 hours. At the same time, all recorded O2•− levels for time period of salt treatments were above control level. These results are compatible with the idea that productions of ROS are low under normal growth condition (as control in the current study) and there is equilibrium between ROS production and defense pathways (such as SOD). Stress conditions such as salt, drought, cold, heavy metals, ultraviolent etc. disturb this cellular homeostasis and enhance the production of ROS (Wang et al. Citation2009) and APX, CAT and SOD are substantial components of ROS-scavenging mechanism of plants (Bowler et al. Citation1992, Asada and Takahashi Citation1987, Polle Citation2001).
Accumulation of ROS including O2•− could act as an oxidative damaging factor at cellular level. A protective or signaling factor depends on the equilibrium between ROS production and scavenging or defense mechanisms, the significant ones being SODs, a family of metalloenzymes catalyzing the dismutation of O2 − to H2O2. Plants can scavenge altered formation of ROS by activation of SOD (Smirnoff Citation1993, Mittler Citation2002, Dat et al. Citation2000). O2•− productions were altered depending on stress application and exposure time of the stress. In the view of this information, decreased orientations of O2•− productions in salt treated plants and stationary orientations of O2•− productions in drought-treated plants can conclude as plants can successfully cope with long time period of salt but not the drought stress. Therefore, the decreased production of O2•− after long time period of salt stress could indicate the activated SOD isoenzymes as a component of antioxidant defense mechanism.
Cold treatment
Tomato plants were exposed to 4°C for the cold stress and samples were collected in different time periods (control, 2 days, 4 days, 6 days, 8 days and 10 days). The O2•− concentrations in cold-treated tomato plants are presented in . Throughout the time period of cold stress O2•− production increased except for other 10 days. While O2•− production had slightly increased in the first 2 days, it reached maximum level after 4 days. This level became constant for 6 days. O2•− production was gradually declined and it was under the control level after 10 days of cold exposure. These results are agreed with the accumulation of ROS under cold stress which has been reported in several plants (Prasad et al. Citation1995, Gonzales-Meler et al. Citation1999). The production of O2•− after 10 days cold exposure which is under the control level can be explained as cell death and interrupted stress perception in tomato tissues.
Heavy metal treatment
Heavy metals are significant environmental contaminants, and their presence in the atmosphere, soil and water can cause serious problems to all organisms. Inhalation and ingestion are the primary routes for heavy metals to enter the human body. Ingestion is the main route of exposure to these heavy metals. Human populations’ intake of heavy metals is through food chain. Vegetables uptake metals by absorbing them from contaminated atmosphere, soil and water (Ellen et al. Citation1990). The bioaccumulation of heavy metal in the food chain can be highly dangerous to human health. Unlike organic compounds, they cannot be degraded or destroyed under biotic conditions. Since there is no good mechanism for their elimination, the chronic low-level intakes of these elements have damaging effects on human beings and other animals. These metals cause environmental hazards and are reported to be exceptionally toxic (Conte et al. Citation1998).
This study was performed to detect the effects of the heavy metal Cd2+ ,Pb2+ , Cu2+ , Mn1+ on the tomato plants utilizing the CMC–G–SOD biosensor to compare the alterations in O2•− concentration. Different metals and concentrations were used to determine the impact of heavy metal treatment on O2•− in tomato plants. For this purpose, 25-day-old plants grown in controlled media were used for stress treatments. The heavy metal stress was performed with Cd2+ , Pb2+ , Cu2+ , Mn1+ with different concentrations for a period of 24 hours. The concentrations for all heavy metals were 0 (control), 80, 160, 320, 640 and 1280 mM. Each metal had a different impact on O2•− enhancement in tomato plants. The O2•− concentration was gradually increased with increasing Cd2+ concentrations (). Maximum production of O2•− was observed at 640 mM Cd2+ treatment. It can be seen from that this production was decreased with the treatment of 1280 mM Cd2+ , but still significantly upon control level.
Figure 5. Effect of Cd+2 concentration on O2•− production in tomato plants after 24 hours treatment.
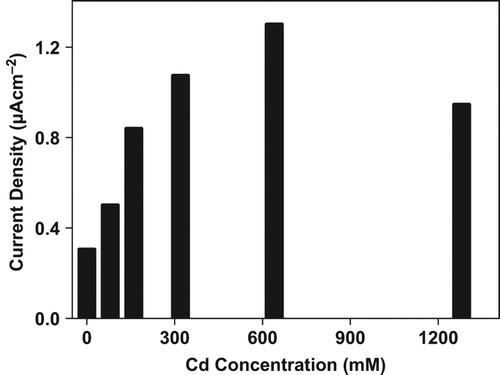
Tomato plants exposed to several concentrations of Pb2+ and Cu2+ contamination resulted in increased O2•− production up to 320 mM treatments of these metal. After 320 mM concentration a gradual decline was observed in O2•− production for both heavy metals ( and ). All patterns of current densities in O2•− production were similar among Cd2+, Pb2+ , Cu2+ contaminated tomato plants. Initially the production of O2•− increased with increasing metal concentration and was followed by a decrease in O2•− production, still protecting their levels at 1280 mM concentration.
Figure 6. Effect of Pb+2 concentration on O2•− production in tomato plants after 24 hours treatment.
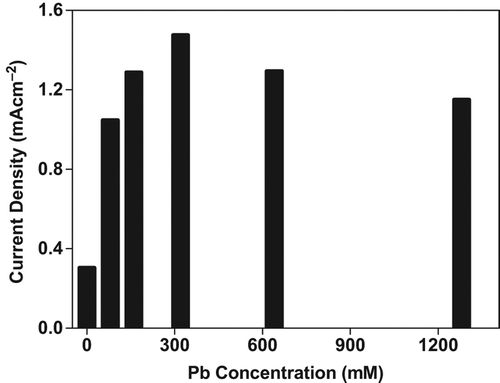
Figure 7. Effect of Cu+2 concentration on O2•− production in tomato plants after 24 hours treatment.
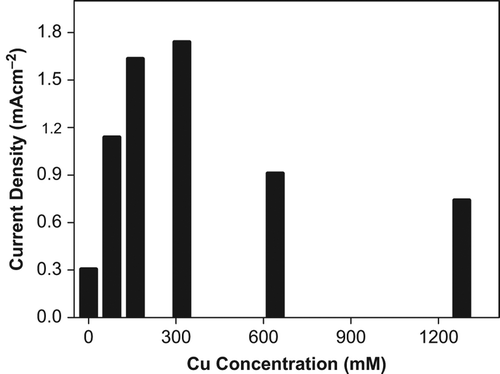
On the other hand, the O2•− production showed alteration with Mn1+ contamination. The O2•− production increased with increasing concentration of Mn1+ up to 1280 mM (). No decreased orientation with the exposure of Mn1+ to the tomato plants was observed. We concluded that with all O2•− production data obtained from tomato plants contaminated with different concentrations of Cd2+, Pb2+, Cu2+ and Mn1+ treatment antioxidant defense mechanism is not stimulated with low concentration of metal contamination. Antioxidant defense mechanisms start to prevent O2•− production and scavenge O2•− at 320 mM concentration for Pb2+ and Cu2+ contamination and 640 mM concentration for Cd2+ contamination. However it could not prevent or scavenge O2•− production in tomato plants exposed to Mn1+ solutions or the rate of O2•− production was significantly higher than scavenge capacity of antioxidant defense mechanism. The other conviction of us about data of O2•− production under Mn1+ contaminations is that the tomato plants is principally sensitive to contamination of this metal solution. The O2•− production increase of 89.6%, 82.5%, 79.4%, 76.6% for Mn1+, Cu2+, Pb2, Cd2+, respectively, was observed by increasing the heavy metal concentration.
Figure 8. Effect of Mn + 1 concentration on O2•− production in tomato plants after 24 hours treatment.
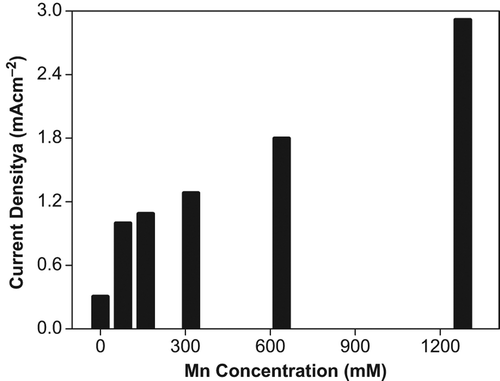
In the current study, O2•− production resulting under different concentrations of Cd2+, Pb2+ , Cu2+, Mn1+ indicate that these heavy metals can substantially intensify the accumulation of ROS in leading to oxidative stress followed by superoxide anion in mitochondria and other organelles of plants (Prasad et al. 1999, Cuypers et al. Citation1999).
Conclusion
A highly sensitive SOD biosensor for the simultaneous determination of superoxide radicals was developed by immobilization of superoxide dismutase within CMC–G on a Pt electrode surface (Kocabay et al. Citation2012). The response of the CMC–G–SOD biosensor was proportional to O2•− concentration and the detection limit was 1.25 × 10‐3 mM with a correlation coefficient of 0.9994 at a signal-to-noise ratio of 3. The response time of the developed biosensor was 2 seconds. The developed biosensor exhibited high analytical performance with wider linear range, high sensitivity and low response time (Kocabay et al. Citation2012). In this study, the application of developed CMC–G–SOD biosensor to agriculture was studied. For this purpose, tomato plants were exposed to heavy metal, cold, salinity and drought stress to examine the O2•− enhancement. The effect of heavy metal, cold, salinity and drought stress in tomato plants on O2•− enhancement was investigated using developed CMC–G–SOD biosensor.
Declaration of interest
The authors report no conflicts of interest. The authors alone are responsible for the content and writing of the paper.
This work financially supported by the Scientific and Technological Research Council of Turkey (no. 108T131)
References
- Allen R. 1995. Dissection of oxidative stress tolerance using transgenic plants. Plants Pysiol. 107:1049–1054.
- Asada K, Takahashi M. 1987. Production and scavenging of active oxygen in photosynthesis. In: Kyle DJ, Osmond CB, Arntzen CJ, et al., Eds.Photoinhibition. Amsterdam: Elsevier. pp. 227–287.
- Auchere F, Rusnak F. 2002. “What is the ultimate fate of superoxide anion in vivo?”. J Biol Inorg Chem. 7:664–667.
- Beck EG, Fettig S, Knake C, Gartig K, Bhattarai T. 2007. Specific and unspecific responses of plant to cold and drought stress. J Biosci. 32:501–510.
- Beissenhirtz MK, Scheller FW, Viezzoli MS, Lisdat F. 2006. Engineered superoxide dismutase monomers for superoxide biosensor applications. Anal Chem. 78:928–935.
- Bowler C, Van Montagu M, Inzé D. 1992. Superoxide dismutase and stress tolerance. Annu Rev Plant Physiol Plant Mol Biol. 43:83–116.
- Carey FA, Sundberg RJ. 1984. Advanced Organic Chemistry, 2nd ed. New York: Plenum.
- Conte C, Mutti I, Puglisi P, Ferrarini A, Regina GRG, Maestri E, Marmiroli N. 1998. DNA fingerprinting analysis by a PCR based method for monitoring the genotoxic effects of heavy metals pollution. Chemosphere. 37:2739–2749.
- Cooper JM, Greenough KR, McNeil CJ. 1993. Direct electron transfer between immobilized cytochrome c and modified gold electrodes. J Electroanal Chem. 347:267–275.
- Cuypers A, Vangronsveld J, Clijsters H. 1999. The chemical behavior of heavy metals plays a prominent role in the induction of oxidative stress. Free Rad Res. 31:839–843.
- DalCorso G, Farinati S, Maistri S, Furini A. 2008. How plants cope with cadmium: staking all on metabolism and gene expression. J Integr Plant Biol. 50:1268–1280.
- Dat J, Vandenabeele S, Vranová E, Van Montagu M, Inze D, Van Breusegem F. 2000. Dual action of the active oxygen species during plant stress responses. Cell Mol Life Sci. 57:779–795.
- Dean RT, Fu S, Stocker R, Davies MJ. 1997. Biochemistry and pathology of radical-mediated protein oxidation. Biochem J. 324:1–18.
- Del Río LA, Lyon DS, Olah I, Glick B, Salin ML. 1983. Immunocytochemical evidence for a peroxisomal localization of manganese superoxide dismutase in leaf protoplasts from a higher plant. Planta. 158:216–224.
- Di J, Bi S, Zhang M. 2004. Third-generation superoxide anion sensor based on superoxide dismutase directly immobilized by sol-gel thin film on gold electrode. Biosensors Bioelectron. 19:1479–1486.
- Di J, Peng S, Shen C, Gao Y, Tu Y. 2007. One-step method embedding superoxide dismutase and gold nanoparticles in silica sol-gel network in the presence of cysteine for construction of third- generation biosensor. Biosensors Bioelectron. 23:88–94.
- Droillard MJ, Paulin A. 1990. Isozymes of superoxide dismutase in mitochondria and peroxisomes isolated from petals of carna- tion (Dianthus caryophyllus) during senescence. Plant Physiol. 94:1187–1192.
- Ellen G, Loon JW, Tolsma K. 1990. Heavy metals in vegetables grown in the Netherlands and in domestic and imported fruits. Z Lebensm Unters Forsch. 190:34–39.
- Finkelstein RR, Gampala SSl, Rock CD. 2002. Absisic acid signaling in seeds and seedlings. Plant Cell. 14:15–45.
- Foye CH, Descourvieres P, Kunert KJ. 1994. Protection against oxygen radicals: an important defense mechanism studied in transgenic plants. Plant Cell Environ. 17:507–523.
- Fridovich I. 1970. Quantitative aspects of production of superoxide anion radical by milk xanthine oxidase. J Biol Chem. 245:4035.
- Fridovich I. 1972. Superoxide radicals and superoxide dismutase. Acc Chem Res. 5:321–326.
- Fridovich I. 1995. Superoxide radicals and superoxide dismutases. Annu Rev Biochem. 64:97–112.
- Fujita M, Tsuruta R, Kasaoka S, Fujimoto K, Tanaka R, Oda Y, et al. 2009. In vivo real-time measurement of superoxide anion radical with a novel electrochemical sensor. Free Radic Biol Med. 47:1039–1048.
- Gonzales-Meler MA, Ribas-carbo M, Giles L, Siedow JN. 1999. The effect of growth and measurement temperature on the activity of the alternative respiratory pathway. Plant Physiol. 120:765–772.
- Gratao PL, Polle A, Lea PJ, Azevedo RA. 2005. Making the life of heavy metal-stressed plants a little easier. Funct Plant Biol. 32:481–494.
- Halliwell B, Gutteridge JMC. 1981. Formation of thiobarbituric-acid-reactive substance from deoxyribose in the presence of iron salts: the role of superoxide and hydroxyl radicals. FEBS Lett. 128:347.
- Halliwell B, Gutteridge JMC. 1984. Oxygen toxicity, oxygen radicals, trasition metals and desease. Biochem J. 219:1–14.
- Halliwell B, Gutteridge JMC. 1986. Oxygen free radicals and iron in relation to biology and medicine: some problems and concepts. Arch Biochem Biophys. 246:501–514.
- Hammond-Kosack KE, Jones JDG. 1996. Resistance gene-dependent plant defense responses. Plant Cell. 8:1773–1791.
- Hensley K, Floyd RA. 2002. Reactive oxygen species and protein oxidation in aging: a look back, a look ahead. Arch Biochem Biophys. 397:377–383.
- Klug D, Rabani J, Fridovich I. 1972. A direct demonstration of the catalytic action of superoxide dismutase through the use of pulse radiolysis. J Biol Chem. 247:4839.
- Kocabay O, Emregul E, Aras S, Emregul KC. 2012. Carboxymethylcellulose-gelatin superoxidase dismutase electrode for amperometric superoxide radical sensing. Bioprocess Biosyst Eng. 35:923–930.
- Land EJ, Swallow AJ. 1971. One-electron reactions in biochemical systems as studied by pulse radiolysis. V. Cytochrome c. Arch Biochem Biophys. 145:365–372.
- Lawrence GD, Sawyer DT. 1979. Potentiometric titrations and oxidation-reduction potentials of manganese and copper-zinc superoxide dismutases. Biochemistry. 18:3045.
- Lisdat F, Ge B, Ehrentreich-Förster E, Reszka R, Scheller FW. 1999. Superoxide dismutase activity measurement using cytochrome c-Modified electrode. Anal Chem. 71:1359–1365.
- Mahajan S, Tuteja N. 2005. Cold, salinity and drought stresses: an overview. Arch Biochem Biophys. 444:139–158.
- Mannino S, Buratti S, Cosio MS, Pellegrini N. 1999. Evaluation of the ‘antioxidant power’ of olive oils based on a FIA system with amperometric detection. Analyst. 124:1115–1118.
- Mc Kersie BD, Leshem YY. 1994. Salt Stress. Stress and Stress Coping in Cultivated Plants. Netherlands: Kluwer Academic Publishers, pp. 55–78.
- McNeil CJ, Smith KA, Bellavite P, Bannister JV. 1989. Application of the electrochemistry of cytochrome c to the measurement of superoxide radical production. Free Radical Res Commun. 7:89.
- Mittler R. 2002. Oxidative stress, antioxidants, and stress tolerance. Trends Plant Sci. 9:405–410.
- Moscone D, Mascini M. 1988. Determination of superoxide dismutase activity with an electrochemical oxygen probe. Anal Chim Acta. 211:195–204.
- Oshaka T, Shintane Y, Matsumoto F, Okajima T, Tokuda K. 1995. Mediated electron transfer of polyethylene oxide-modified superoxide dismutase by methyl viologen. Bioelectrochem Bioenerget. 37:73.
- Paliyath G, Pinhero RG, Rao MV, Murr DP, Fletcher RA. 1997. Changes in activities of antioxidant enzymes and their relationship to genetic and paclobutrazol-induced chilling tolerance in maize seedlings. Plant Physiol. 114:695–704.
- Parent B, Hachez C, Redondo E, Simonneau T, Chaumont F, Tardieu F. 2009. Drought and absisic acid effects on aquaporin content translate into changes in hydraulic conductivity and leaf growth rate: atrans-scale approach. Plant Phsiol. 149:2000–2012.
- Pinzino C, Capocchi A, Galleschi L, Saviozzi F, Nanni B, Zandomeneghi M. 1999. Aging, free radicals, and antioxidants in wheat seeds. J Agric Food Chem. 47:1333–1339.
- Polle A. 2001. Dissecting the superoxide dismutase-ascorbate peroxidase-glutathione pathway in chloroplasts by metabolic modeling. Computer simulations as a step towards flux analaysis. Plant Physiol. 126:445–462.
- Prasad K, Kalra J, Bhardwaj B. 1989. Increased chemiluminescence of polymorphonuclear leucocytes in dogs with volume overload heart failure. Br J Exp Pathol. 70:463.
- Prasad KVSK, ParadhaSaradhi P, Sharmila P. 1999. Concerted action of antioxidant enzymes and curtailed growth under zinc toxicity in Brassica juncea. Environ Exp Bot. 42:1–10.
- Prasad TK, Anderson MD, Stewart CR. 1995. Localization and characterization of peroxidases in the mitocindria of chilling-acclimated maize seedlings. Plant Physiol. 108:1597–1605.
- Raghavendra AS, Gimono J, Van Deynze A, Walia H, Blumwald E. 2010. ABA perception and signaling. Trends Plant Sci. 15:395–401.
- Rigo A, Viglino P, Rotilio G. 1992. Polarographic determination of superoxide dismutase. Anal Biochem. 68:1–8.
- Rotilio G, Bray RC, Fielden EM. 1972. A pulse radiolysis study of superoxide dismutase. Biochim Biophys Acta. 268:605.
- Salin ML, Bridges SM. 1980. Isolation and characterization of an iron‐containing superoxide dismutase from a eucaryote, Brassica campestris. Arch Biochem Biophys. 201:369–374.
- Scandalios JG. 1993. Oxygen stress and superoxide dismutases. Plant Physiol. 101:7–12.
- Smirnoff N. 1993. The role of active oxygen in response of plants to water deficit and dessication. New Phytologist. 125:27–58.
- Tammeveski K, Tenno TT, Mashirin AA, Hillhouse EW, Manning P, McNeil CJ. 1998a. Superoxide electrode based on covalently immobilized cytochrome c: modelling studies. Free Radical Res Commun. 25:973–978.
- Tammeveski K, Tenno TT, Mashirin AA, Hillhouse EW, Manning P, McNeil CJ. 1998b. Superoxide electrode based on covalently immobilized cytochrome c: modelling studies. Free Radic Biol Med. 25:973.
- Tanaka K, Muto Y. 1992. Amperometric determination of superoxide anions generated from phytoplankton Chattonella antique. Bioelectrochem Bioenerg. 29:143.
- Tian T, Wang H, Abdallah AM, Prinyawiwatkul W, Xu Z. 2011. Red and White wines inhibit cholesterol oxidation induced by free radicals. J Agr Food Chem. 59:6453–6458.
- Van Camp W, Willekens H, Bowler C, Van Montagu M, Inze D, Langebartels C, Sandermann H. 1994. Elevated levels of superoxide dismutase protect transgenic plants against ozone damage. Bio Technol. 12:165–168.
- Van Lente F. 1993. Free radicals. Anal Chem. 65:374–377.
- Verma OP, Santoshi US, Srivastava HK. 2003. Governance of gene action and combining ability for certain grain quality traits in three diverse rice (Oryza sativa L.) growing ecosystems. J Sustain Agr. 22:63–78.
- Verma S, Dubey RS. 2003. Lead toxicity induces lipid peroxidation and alters the activites of antioxidant enzymes in growing rice plants. Plant Sci. 164:645–655.
- Wang Y, Wu Y, Wang J, Di J. 2009. Disposable superoxide anion biosensor based on superoxide dismutase entrapped in silica sol-gel matrix at gold nanoparticles modified ITO electrode. Bioprocess Biosyst Eng. 32:531–536.
- Willekens H, Chamnongpol S, Davey M, Schrauder M, Langebartels C, Van Montagu M, et al. 1997. Catalase is a sink for H2O2 and is indispensable for stress defence in C-3 plants. EMBOJ. 16:4806–4816.
- Youdim KA, Joseph JA. 2001. A possible emerging role of phytochemicals in improving age-related neurological dysfunctions: a multiplicity of effects. Free Radical Biol Med. 30:583–594.
- Zhu JK. 2001. Cell signaling under salt, water and cold stresses. Curr Opin Plant Biol. 4:401–406.