Abstract
Context: Andrographolide, extracted from the leaves of Andrographis paniculata (Burm. f.) Nees (Acanthaceae), is a labdane diterpene lactone. It is widely reported to possess anti-inflammatory and antitumorigenic activities. Cerebral endothelial cells (CECs) play a crucial role in supporting the integrity and the function of the blood–brain barrier (BBB). However, no data are available concerning the effects of andrographolide in CECs. The aim of this study was to examine the detailed mechanisms of andrographolide on CECs.
Objective: This study investigated a novel bioactivity of andrographolide on cerebral ischemia/reperfusion-induced brain injury.
Materials and methods: CECs were treated with andrographolide (20–100 µΜ) for the indicated times (0–24 h). After the reactions, cell survival rate and cytotoxicity were tested by the MTT assay and the lactate dehydrogenase (LDH) test, respectively. Western blotting was used to detect caspase-3 expression. In addition, analysis of cell cycle and apoptosis using PI staining and annexin V-FITC/PI labeling, respectively, was performed by flow cytometry. We also investigated the effect of andrographolide on middle cerebral artery occlusion (MCAO)/reperfusion-induced brain injury in a rat model.
Results: In the present study, we found that andrographolide (50–100 µΜ) markedly inhibited CEC growth according to an MTT assay and caused CEC damage according to a LDH test. Our data also revealed that andrographolide (50 µM) induced CEC apoptosis and caspase-3 activation as respectively detected by PI/annexin-V double staining and western blotting. Moreover, andrographolide arrested the CEC cell cycle at the G0/G1 phase by PI staining. In addition, andrographolide (5 mg/kg) caused deterioration of MCAO/reperfusion-induced brain injury in a rat model.
Conclusions: These data suggest that andrographolide may disrupt BBB integrity, thereby deteriorating MCAO/reperfusion-induced brain injury, which are, in part, associated with its capacity to arrest cell-cycle and induce CEC apoptosis.
Introduction
The brain endothelium constitutes a barrier to the passive movement of substances from the blood into the cerebral microenvironment (Fraser, Citation2011). In addition to regulating vasomotor tone, platelet adhesion and neovascularization, cerebral endothelial cells (CECs) play a crucial role in supporting the integrity and the function of the blood–brain barrier (BBB) (Rizzo & Leaver, Citation2010). However, CEC apoptosis was suggested to disrupt the BBB integrity, subsequently leading to brain edema and neuronal damage (Rizzo & Leaver, Citation2010). Cheng et al. (Citation2003) demonstrated that ischemia-induced apoptosis of CECs was mediated by increased expression of p53 and activation of caspase-3 signaling. Deposition of β-amyloid (Aβ) also induced CEC death via caspase-8/reactive oxygen species (ROS)/apoptosis signal-regulating kinase 1 (ASK)1-dependent apoptosis (Hsu et al., Citation2007; Xu et al., Citation2001). Therefore, protection of the BBB from apoptosis may be a beneficial therapeutic strategy for neuronal functions.
Apoptosis or programmed cell death, typically occurring in nucleated cells, is a form of cellular suicide that is essential for general development and tissue homeostasis in all metazoan organisms. Profound alteration of the cellular architecture characterizes apoptotic cell death, including cell shrinkage, membrane blebbing, DNA fragmentation, chromatin condensation and phosphatidylserine (PS) exposure (Mills et al., Citation1999). In humans, apoptotic deregulation can lead to diseases such as cancer, immune disorders and neurodegenerative diseases (Green & Evan, Citation2002; Yuan & Yankner, Citation2000).
Andrographolide, a labdane diterpene lactone, is the most active and important constituent isolated from the leaves of Andrographis paniculata (Burm. f.) Nees (Acanthaceae) (Coon & Ernst, Citation2004). Recent studies demonstrated that andrographolide possesses anticancer, anti-inflammatory and hepatoprotective activities (Bao et al., Citation2009; Negi et al., Citation2008). Recently, we also demonstrated that andrographolide enhanced nuclear factor (NF)-κB subunit p65 Ser536 dephosphorylation through the activation of protein phosphatase 2A in vascular smooth muscle cells (Hsieh et al., Citation2011) and that andrographolide possesses anti-platelet activity via regulating the p38 MAPK-hydroxyl radical-ERK2 cascade (Lu et al., Citation2011, Citation2012). In addition, andrographolide also inhibited endothelial cell apoptosis through activation of the phosphatidylinositol-3kinase (PI3K)/Akt pathway (Chen et al., Citation2004), and blocked tumor necrosis factor (TNF)-α-mediated intercellular adhesion molecule 1 (ICAM-1) expression and NF-κB activation in a human endothelial cell line (Chao et al., Citation2011). On the contrary, andrographolide has pro-apoptotic activities in a variety of tumor cell lines (Li et al., Citation2007). Moreover, in a recent study, we also demonstrated that andrographolide could induce platelet apoptosis through the extrinsic apoptotic pathway, including caspase-8 activation and mitochondrial depolarization (Lien et al., Citation2012). This evidence revealed andrographolide’s multiple regulation in different types of cells.
However, the cerebral vasculature possesses unique morphological and functional features compared to endothelial cells derived from vessels of peripheral organs (Rizzo & Leaver, Citation2010). Although, as described above, andrographolide has anti-inflammatory and anti-apoptotic actions in endothelial cells, the effects of andrographolide on CECs still remain unknown. Therefore, for the first time, we examined the detailed mechanisms underlying andrographolide’s signaling pathways in CECs.
Materials and methods
Materials
Andrographolide (≥98%, Mw. 350.45), 3-(4,5-dimethylthiazol-2-yl)-2,5-diphenyltetrazolium bromide (MTT) and 2,3,5-triphenyltetrazolium (TTC) were from Sigma (St. Louis, MO; the anti-cleaved caspase-3 monoclonal antibody (mAb) was from Cell Signaling (Beverly, MA); the anti-α-tubulin mAb was from NeoMarkers (Fremont, CA); Fluor 488 annexin V and propidium iodide (PI) kit was from Molecular Probes (Eugene, OR) and the LDH-Cytotoxicity assay kit was from Biovision (Mountain View, CA). Andrographolide was dissolved in 0.5% dimethyl sulfoxide (DMSO) and stored at 4 °C until used.
Mouse CEC primary culture
Mouse CECs were prepared as described previously (Hsu et al., Citation2007). Briefly, the gray matter of fresh mouse brains was homogenized and filtered, and the resulting fraction was then sequentially digested with 4 mg/mL collagenase B for 2 h and 1 mg/mL collagenase/dispase (Roche Molecular Biochemicals, Indianapolis, IN) for 2 h, followed by centrifugation in a 40% Percoll solution. The second band containing the microvessels was collected and washed before plating onto collagen-coated dishes. CECs migrating from isolated microvessels were maintained in Dulbecco's modified Eagle's medium (DMEM), with high glucose and L-glutamine supplemented with 10% fetal bovine serum (FBS), cell cultures (between passages 4 and 15) were grown to 85–95% confluence before use. Mouse primary CECs were uniformly positive for factor VIII, vimentin and characteristic bradykinin receptors (at 95% endothelial cell purity).
Cell viability assay
Cell viability was measured by a colorimetric MTT assay and LDH assay. Briefly, cells (3 × 104 cells/well) were cultured in 24-well plates and incubated with the vehicle or various concentrations (5–100 µM) of andrographolide for 24 h. After treatment, MTT (1 mg/mL) was added and further incubated for 1 h. Then cells were lysed in 400 µL DMSO. In the LDH assay, the LDH reagent was added to the supernatants and incubated for 10 min at room temperature. The absorbance was measured at 550 nm on a microplate reader. Each experiment was performed in triplicate and repeated at least three times. The percentage of MTT was compared to the solvent control (DMSO) group, and the percentage of LDH release was calculated using the formula of the LDH assay kit (BioVision).
Flow cytometric analysis
Cell cycle and apoptosis were determined by PI staining and annexin V-FITC/PI labeling, respectively, according to the manufacturer’s protocol. Briefly, cells (6 × 105 cells/mL) were pretreated with andrographolide (5–100 μM) or a solvent control (0.1% DMSO) for 24 h. After incubation with dyes for 30 min, cells were then assayed using a flow cytometer (Beckman Coulter, Miami, FL). Data were collected from 10 000 cells per experimental group, and appropriate scatter gates were set to exclude cellular debris. All experiments were repeated at least four times to ensure reproducibility.
Immunoblotting study
Cells (6 × 105 cells/well) were preincubated with andrographolide (50 μM) or a solvent control (0.1% DMSO) for the indicated time intervals (0–2 h). After the reaction, cells were immediately re-suspended in 100 μl of lysis buffer. Samples containing 50 μg of protein were separated by a 14% sodium dodecylsulfate polyacrylamide gel electrophoresis (SDS-PAGE); proteins were electrotransferred by semidry transfer (Bio-Rad, Hercules, CA). Blots were blocked with TBST (10 mM Tris-base, 100 mM NaCl, and 0.01% Tween 20) containing 5% bovine serum albumin (BSA) for 1 h and then probed with an anti-cleaved caspase-3 primary antibody. Membranes were incubated with horseradish peroxidase (HRP)-linked anti-mouse immunoglobulin G (IgG; diluted 1:3000 in TBST) for 1 h. Immunoreactive bands were detected by an enhanced chemiluminescence (ECL) system. The bar graph depicts the ratios of semiquantitative results obtained by scanning reactive bands and quantifying the optical density using videodensitometry (Bio-profil; Biolight Windows Application V2000.01; Vilber Lourmat, France).
Middle cerebral artery occlusion-induced transient focal cerebral ischemia in rats
Male Wistar rats (250–300 g) were used in this study. All animal experiments and care were performed according to the Guide for the Care and Use of Laboratory Animals (National Academy Press, Washington, DC, 1996). Before undergoing the experimental procedures, all animals were confirmed to be clinically normal, were free of apparent infection or inflammation and showed no neurological deficits, as evaluated by a spontaneous rotational test.
Animals were anesthetized with a mixture of 75% air and 25% O2 gases containing 3% isoflurane. The rectal temperature was maintained at 37 ± 0.5 °C. The right middle cerebral artery (MCA) was occluded as described in our previous report (Hsiao et al., Citation2005). Briefly, the right common carotid artery was exposed, and a 4-0 monofilament nylon thread (25 mm) coated with silicon was inserted from the external to the internal carotid artery until the tip occluded the origin of the MCA. After closure of the operative sites, the animals were allowed to awake from the anesthesia. During another brief period of anesthesia, the filament was gently removed after 1 h of middle cerebral artery occlusion (MCAO). An observer blinded to the identity of the groups assessed the neurological deficits at 1 and 24 h after reperfusion (before euthanization) by a forelimb akinesia (also called the postural tail-hang) test, whereas a spontaneous rotational test was used as a criterion for evaluating the ischemic insult (Lee et al., Citation2002). Animals not showing behavioral deficits at the above time points after reperfusion were excluded from the study. On the other hand, reperfusion was also ensured by an improvement in the ipsilateral local blood flow to at least 60% of the baseline following an initial sharp decrease to about 50–60% of the baseline caused by MCAO as determined using a continuous laser Doppler flow meter (LDF; Oxford Array™, Oxford Optronix, Oxford, UK) with a standard needle probe (pp-051).
Rats were euthanized by decapitation after 24 h of reperfusion. The brains were cut into 2 mm coronal slices starting 1 mm from the frontal pole. Each stained brain slice (stained with 2% TTC) was drawn using a computerized image analyzer (Image-Pro plus, Rockville, MD). The calculated infarct areas were compiled to obtain the infarct volume (mm3) per brain. Infarct volumes were expressed as a percentage of the contralateral hemisphere volume using the formula: (area of the intact contralateral [left] hemisphere – area of the intact region of the ipsilateral [right] hemisphere) to compensate for edema formation in the ipsilateral hemisphere (Hsiao et al., Citation2005).
All animals were divided into three groups: (1) a sham-operated group; (2) a solvent (DMSO)-treated group, and (3) a group treated with a single dose of 5 mg/kg, i.p. of andrographolide. Rats received the isovolumetric normal saline, solvent or andrographolide (5 mg/kg) 10 min before MCAO was performed.
Data analysis
The experimental results are expressed as the means ± S.E.M. and are accompanied by the number of observations. All experiments were assessed by an analysis of variance (ANOVA). If this analysis indicated significant differences among group means, then each group was compared using the Newman–Keuls method. p < 0.05 was considered statistically significant.
Results
Effects of andrographolide on the cell viability of CECs
In the present study, we found that andrographolide (20–100 µM) decreased cell viability of CECs in a dose-dependent manner, as demonstrated by a MTT test (). Moreover, we also performed a cytotoxicity test (LDH release). As shown in , treatment with andrographolide (50 and 100 µM) for 24 h notably increased LDH release by 27.9 ± 5.0 and 39.6 ± 2.8%, respectively. In addition, drastic morphological changes were also observed in the group of cells treated with 50 µM andrographolide by bright-field microscopy (), which suggests cell death has occurred.
Figure 1. Effects of andrographolide on cell viability and cytotoxicity. CECs were incubated with andrographolide (5–100 μM) or 0.1% DMSO for 24 h, and then cell viability and cytotoxicity were determined by an MTT assay (A) and lactate dehydrogenase (LDH) test (B), respectively. (C) Morphological changes were observed by bright-field microscopy at a magnification of 40×. Data in (A, B) are presented as the means ± S.E.M. (n = 3). *p < 0.05, **p < 0.01, and ***p < 0.001, compared to the solvent control (DMSO) group. The profiles (C) are representative examples of four similar experiments.
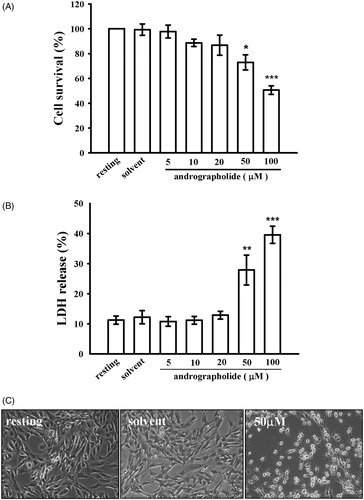
Effects of andrographolide on CEC apoptosis
To determine whether andrographolide could stimulate CEC apoptosis, annexin-V and PI double staining was used to define cell apoptosis by flow cytometry. We found that treatment with andrographolide (50 and 100 μM) for 24 h induced CEC apoptosis compared to the solvent control (25.2 ± 4.8 and 46.2 ± 4.2% versus 6.7 ± 1.0%) ().
Figure 2. Effects of andrographolide on cell apoptosis. (A) CECs were incubated with andrographolide (5–100 μM) or 0.1% DMSO for 24 h, and then cell apoptosis was detected by annexin-V/PI double staining. The lower panel shows the statistical analysis of the percentage of apoptotic cells. (B) Activation of caspase-3, a marker of apoptosis, was also measured at the indicated time intervals after 50 μM andrographolide treatment by western blotting with an anti-caspase-3 antibody. Data are presented as the means ± S.E.M. (n = 3). *p < 0.05, **p < 0.01, and ***p < 0.001, compared to the solvent control (DMSO) group.
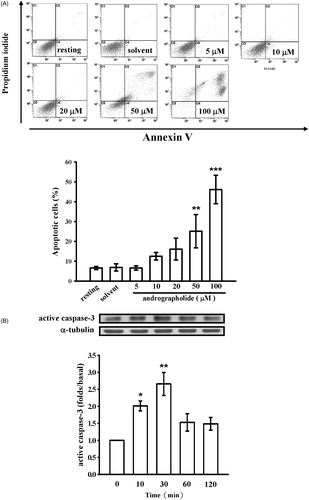
In addition, activation of caspase-3 (a critical marker of apoptosis) was also determined. As shown in , andrographolide (50 μM) time-dependently triggered caspase-3 activation, indicating that andrographolide, indeed, could induce cell apoptosis, eventually leading to cell death or cell necrosis in the late stage.
Effects of andrographolide on CEC growth
In the present study, PI staining was used to analyze whether andrographolide was involved in regulating cell cycle of CECs. As shown in , andrographolide (30 and 40 μM) at 24 h markedly increased the cell distribution in the G0/G1 phase by 26.4 ± 3.1 and 39.9 ± 3.0%, respectively, which suggests that andrographolide may arrest cell growth in the G0/G1 phase and impede cell entry into the S or G2/M phase, ultimately inhibiting cell growth.
Figure 3. Effects of andrographolide on the cell cycle. (A) CECs were treated with andrographolide (5–100 μM) or 0.1% DMSO for 24 h, and harvested to assess the cell cycle by staining with PI. Flow cytometric data are presented. (B) Statistical analysis of cells at G0/G1 phase. Data are presented as the means ± S.E.M. (n = 3). ***p < 0.001, compared to the solvent control (DMSO) group.
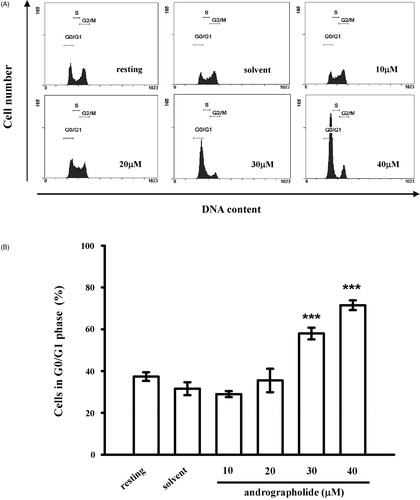
Effects of andrographolide on MCAO-induced focal cerebral ischemia in rats
All animals in this study showed similar physiological values (i.e., rectal temperature and mean arterial blood pressure) before, during and after MCAO among the groups (data not shown). Neither abnormal behavior, depression of respiration, nor hypothermia was observed in the solvent- or andrographolide-treated groups. The cerebral infarction was examined using 2 mm thick slices of the cerebrum 24 h after MCAO reperfusion in rats through TTC staining. shows typical photographs of coronal sections of sham-operated, solvent (DMSO)-treated and andrographolide-treated groups (5 mg/kg) prior to the ischemic insult. Administration of 5 mg/kg andrographolide produced pronounced increases by 15% in the infarct volume (white area) compared to the solvent-treated group.
Figure 4. Effects of andrographolide (andro) on middle cerebral artery occlusion (MCAO)/reperfusion-induced brain injury in rats. Coronal sections of brains were stained by TTC in rats at 24 h after MACO-reperfusion. Cerebral infarction in sham-operated (sham) or MACO-reperfusion rats is from a representative animal that received solvent (DMSO) or andrographolide (5 mg/kg) intraperitoneally.
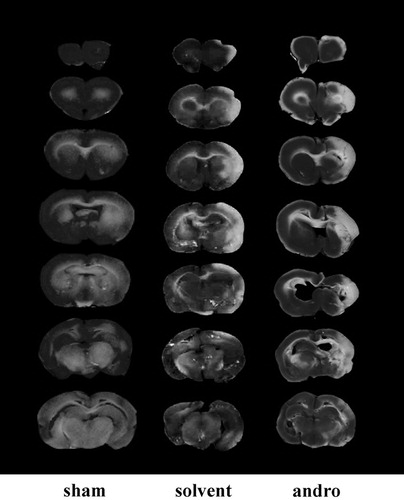
Discussion
In the present study, we demonstrated for the first time that andrographolide deteriorated MCAO/reperfusion-induced brain injury in rats through, at least in part, induction of CEC apoptosis and inhibition of CEC growth (). The dose of andrographolide in clinical therapy for most patients is 60 mg/d, and the maximal dose is 360 mg/d (Coon & Ernst, Citation2004). Suo et al. (Citation2007) studied the pharmacokinetics of andrographolide (10 mg/kg; i.v.) in rats and found that the blood concentration of andrographolide was approximately 11 μg/mL (∼30 μM). At this concentration, andrographolide was reported to possess anti-inflammatory, anti-tumor and anti-thrombotic properties in in vitro studies (Bao et al., Citation2009; Lu et al., Citation2011, Citation2012; Zhou et al., Citation2008). In the present study, our data showed that andrographolide at 50 µM caused CEC death or apoptosis ( and ). Moreover, andrographolide also inhibited CEC growth by arresting the cell cycle at the G0/G1 phase (). Tissue homeostasis is dependent on the proper relationships among cell proliferation, differentiation and cell death, which is strictly maintained by both cell cycle control and apoptosis. Previous studies have reported that cell cycle arrest may lead to cell apoptosis (King & Lidlowski, Citation1998). Andrographolide reportedly arrest cell cycle at the G0/G1 or G2/M phase (Li et al., Citation2007; Yan et al., Citation2012). Therefore, we speculate that andrographolide may arrest CEC cell cycle at the G0/G1 phase, and initiate CEC apoptosis, finally proceeding to the phase of late apoptosis (necrosis). However, several reports revealed that andrographolide can protect endothelial cells from apoptosis and inflammation (Chao et al., Citation2011; Chen et al., Citation2004). Chen et al. (Citation2004) suggests that andrographolide (1–100 µM) prevents entothelial cell apoptosis via activating Akt-Bad pathway. Chao et al. (Citation2011) showed that andrographolide (5–10 µM) can inhibit TNF-α-induced NF-κB activation and ICAM-1 expression. However, these discrepancies in both endothelial cells and CECs subjected to andrographolide might be due to their different morphological and functional features (Hawkins & Davis, Citation2005). In fact, Sharifuddin et al. (Citation2012) also indicated that andrographolide could induce dose-dependent cellular toxicity in human lymphoblastoid cell lines, primarily via necrosis. The authors suggest that andrographolide (30 and 50 µM)-induced the low frequency of apoptosis is possibly due to profound cellular damage and DNA damage as well as disruption to the apoptotic machinery, forcing the cells to follow the necrotic route of cell death in AHH-1 and MCL-5 cells. Therefore, andrographolide may possess cytotoxic effects, at least, in some types of cells, including CECs.
Figure 5. Hypothetical scheme of andrographolide-induced apoptosis of CECs. Andrographolide may induce caspase-3 activation and arrest cell cycle of CECs, followed by induction of CEC apoptosis and subsequent BBB disruption. Finally, andrographolide may lead to brain damage.
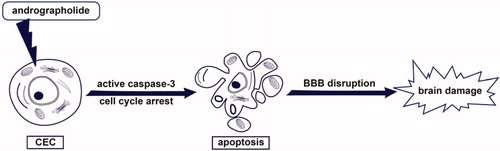
However, CEC apoptosis was suggested to disrupt the BBB integrity, subsequently leading to brain edema and neuronal damage (Rizzo & Leaver, Citation2010). Therefore, a rat model of ischemia/reperfusion-induced brain injury was performed in this study. We found that andrographolide (5 mg/kg) cause deterioration of MCAO/reperfusion-induced brain injury (). It suggests that the deteriorating effect of andrographolide on brain injury, in part, is associated with its capacity to induce CEC apoptosis. On the other hand, previous studies showed that andrographolide at a rather low dose (0.1 mg/kg) could protect neuronal function against inflammation from ischemia/reperfusion-induced brain injury in a rat or mouse model (Chan et al., Citation2010; Chern et al., Citation2011). This evidence indicate that andrographolide at a rather low dose (0.1 mg/kg) protects against brain injury, but it worsens brain injury at a higher dose (5 mg/kg).
Conclusion
Taken together, the most important findings of this study demonstrate for the first time that andrographolde arrests cell cycle of CECs and triggers caspase-3 activation, followed by the induction of CEC apoptosis in vitro, which may deteriorate MCAO/reperfusion-induced brain injury (). Moreover, this study also suggests that surveillance of brain function should be considered when high dose of andrographolide is used to treat patients with inflammatory diseases or cancers.
Declaration of interest
The authors declare they have no conflicts of interest.
Acknowledgements
This work was supported by grants from the National Science Council of Taiwan (NSC100-2320-B-038-021-MY3), the Wan-Fang Hospital-Taipei Medical University (99TMU-WFH-02-2), Chi-Mei Medical Center-Taipei Medical University (99CM-TMU-09) and Shin Kong Wu Ho-Su Memorial Hospital (SKH-8302-101-NDR-09 and SKH-8302-102-NDR-04).
References
- Bao Z, Guan S, Cheng C, et al. (2009). A novel antiinflammatory role for andrographolide in asthma via inhibition of the nuclear factor-kappaB pathway. Am J Respir Crit Care Med 179:657–65
- Chan SJ, Wong WS, Wong PT, Bian JS. (2010). Neuroprotective effects of andrographolide in a rat model of permanent cerebral ischaemia. Br J Pharmacol 161:668–79
- Chao CY, Lii CK, Tsai IT, et al. (2011). Andrographolide inhibits ICAM-1 expression and NF-kappaB activation in TNF-alpha-treated EA.hy926 cells. J Agric Food Chem 59:5263–71
- Chen JH, Hsiao G, Lee AR, et al. (2004). Andrographolide suppresses endothelial cell apoptosis via activation of phosphatidyl inositol-3-kinase/Akt pathway. Biochem Pharmacol 67:1337–45
- Cheng T, Liu D, Griffin JH, et al. (2003). Activated protein C blocks p53-mediated apoptosis in ischemic human brain endothelium and is neuroprotective. Nat Med 9:338–42
- Chern CM, Liou KT, Wang YH, et al. (2011). Andrographolide inhibits PI3K/AKT-dependent NOX2 and iNOS expression protecting mice against hypoxia/ischemia-induced oxidative brain injury. Planta Med 77:1669–79
- Coon JT, Ernst E. (2004). Andrographis paniculata in the treatment of upper respiratory tract infections: A systematic review of safety and efficacy. Planta Med 70:293–8
- Fraser PA. (2011). The role of free radical generation in increasing cerebrovascular permeability. Free Radic Biol Med 51:967–77
- Green DR, Evan GI. (2002). A matter of life and death. Cancer Cell 1:19–30
- Hawkins BT, Davis TP. (2005). The blood–brain barrier/neurovascular unit in health and disease. Pharmacol Rev 57:173–85
- Hsiao G, Lin KH, Chang Y, et al. (2005). Protective mechanisms of inosine in platelet activation and cerebral ischemic damage. Arterioscler Thromb Vasc Biol 25:1998–2004
- Hsieh CY, Hsu MJ, Hsiao G, et al. (2011). Andrographolide enhances nuclear factor-kappaB subunit p65 Ser536 dephosphorylation through activation of protein phosphatase 2A in vascular smooth muscle cells. J Biol Chem 286:5942–55
- Hsu MJ, Hsu CY, Chen BC, et al. (2007). Apoptosis signal-regulating kinase 1 in amyloid beta peptide-induced cerebral endothelial cell apoptosis. J Neurosci 27:5719–29
- King KL, Cidlowski JA. (1998). Cell cycle regulation and apoptosis. Annu Rev Physiol 60:601–17
- Lee EJ, Chen HY, Wu TS, et al. (2002). Acute administration of Ginkgo biloba extract (EGb 761) affords neuroprotection against permanent and transient focal cerebral ischemia in Sprague-Dawley rats. J Neurosci Res 68:636–45
- Li J, Cheung HY, Zhang Z, et al. (2007). Andrographolide induces cell cycle arrest at G2/M phase and cell death in HepG2 cells via alteration of reactive oxygen species. Eur J Pharmacol 568:31–44
- Lien LM, Su CC, Hsu WH, et al. (2012). Mechanisms of andrographolide-induced platelet apoptosis in human platelets: Regulatory roles of the extrinsic apoptotic pathway. Phytother Res in press, doi: 10.1002/ptr.4911
- Lu WJ, Lee JJ, Chou DS, et al. (2011). A novel role of andrographolide, an NF-kappa B inhibitor, on inhibition of platelet activation: The pivotal mechanisms of endothelial nitric oxide synthase/cyclic GMP. J Mol Med (Berl) 89:1261–73
- Lu WJ, Lin KH, Hsu MJ, et al. (2012). Suppression of NF-kappaB signaling by andrographolide with a novel mechanism in human platelets: Regulatory roles of the p38 MAPK-hydroxyl radical-ERK2 cascade. Biochem Pharmacol 84:914–24
- Mills JC, Stone NL, Pittman RN. (1999). Extranuclear apoptosis: The role of the cytoplasm in the execution phase. J Cell Biol 146:703–8
- Negi AS, Kumar JK, Luqman S, et al. (2008). Recent advances in plant hepatoprotectives: A chemical and biological profile of some important leads. Med Res Rev 28:746–72
- Rizzo MT, Leaver HA. (2010). Brain endothelial cell death: Modes, signaling pathways, and relevance to neural development, homeostasis, and disease. Mol Neurobiol 42:52–63
- Sharifuddin Y, Parry EM, Parry JM. (2012). The genotoxicity and cytotoxicity assessments of andrographolide in vitro. Food Chem Toxicol 50:1393–8
- Suo XB, Zhang H, Wang YQ. (2007). HPLC determination of andrographolide in rat whole blood: Study on the pharmacokinetics of andrographolide incorporated in liposomes and tablets. Biomed Chromatogr 21:730–4
- Xu J, Chen S, Ku G, et al. (2001). Amyloid beta peptide-induced cerebral endothelial cell death involves mitochondrial dysfunction and caspase activation. J Cereb Blood Flow Metab 21:702–10
- Yan J, Chen Y, He C, et al. (2012). Andrographolide induces cell cycle arrest and apoptosis in human rheumatoid arthritis fibroblast-like synoviocytes. Cell Biol Toxicol 28:47–56
- Yuan J, Yankner BA. (2000). Apoptosis in the nervous system. Nature 407:802–9
- Zhou J, Lu GD, Ong CS, et al. (2008). Andrographolide sensitizes cancer cells to TRAIL-induced apoptosis via p53-mediated death receptor 4 up-regulation. Mol Cancer Ther 7:2170–80