Abstract
Context: Angelica sinensis (Oliv.) Diels (Apiaceae) polysaccharides (ASP) may play a key role in anti-ischemic activity. However, the anti-atherosclerotic activity and mechanism are unknown.
Objective: This study investigated the protective effects of ASP against ox-LDL-induced senescence of EPCs and explored its underlying molecular mechanisms.
Materials and methods: Mononuclear cells were isolated from bone marrow (BM) of SD rats and differentiated to EPCs. EPCs were exposed to oxidized low-density lipoprotein (ox-LDL, 10 µg/mL, 24 h) and incubated with or without high-dose (100 µg/mL, 48 h) or low-dose (20 µg/mL, 48 h) ASP. Another group of EPCs was pre-treated with Wortmannin (100 nM, 45 min), a PI3K/Akt inhibitor. EPC senescence, telomerase activity, and superoxide anion levels were assessed using SA-β-galactosidase staining, telomerase PCR-ELISA analysis, and DHE staining, respectively. The expression of related proteins, including Akt, p-Akt, hTERT, p-hTERT, and gp91phox, were detected using western blot.
Results: EPCs (47.3%) were SA-β-gal positive after treatment by ox-LDL, additionally, ox-LDL significantly increased superoxide anion levels (375% versus 100%), and inhibited telomerase activity (42% versus 100%). However, the pro-senescent effect of ox-LDL was attenuated about three-fold (16.7%), superoxide anion levels were decreased more than two-fold (148%), and telomerase activity was recovered partly (88% versus 42%) in the EPCs when treated with ASP (100 µg/mL). The immunoblotting confirmed that ASP attenuated inhibition of phosphorylation of Akt and hTERT induced by ox-LDL and down-regulated increased the expression of gp91-phox. Moreover, some effects of ASP were partially abrogated in the presence of Wortmannin.
Discussion: Ox-LDL induced senescence of EPCs via inhibition of telomerase activity, which was influenced by oxidative stress and the Akt/hTERT pathway. The inhibition of EPC senescence by ASP could be important for potential therapeutics.
Conclusion: Treatment of EPCs with ASP remarkably attenuates the harmful effects of ox-LDL via augmentation of Akt/hTERT phosphorylation and inhibition of oxidative stress.
Introduction
Atherosclerosis (AS), or the hardening and narrowing of arteries, is the most common cause of fatal ischemic cardiovascular and cerebrovascular diseases, including myocardial infarction and stroke. These diseases are the leading causes of death worldwide (Libby et al., Citation2011). Endothelial progenitor cells (EPCs) play an essential role in the pathogenesis of AS. Asahara et al. (Citation1997) showed that BM-derived CD34+VEGFR-2+(KDR 2) monocyte cells isolated from human blood and grown in culture were able to differentiate into cells with endothelial cell characteristics. These cells were defined as EPCs. EPCs affect vascular endothelial repair and neovascularization by maintaining vascular function and homeostasis, and inhibiting the formation of atheromatous plaque (Ruan et al., 2013). Based on numerous studies, autologous bone marrow (BM) stem cells and peripheral blood endothelial progenitor cells have been employed in clinics to remedy ischemic diseases (Krankel et al., Citation2012; Lavoie & Stewart, Citation2012).
However, the risk factors for cardiovascular and cerebrovascular diseases, including hypertension, hyperlipidemia, hyperglycemia, age, and smoking, impair the number and the function of circulating EPCs, markedly limiting their therapeutic potential (Raval & Losordo, Citation2013). Cell senescence caused by these pathological factors can result in EPC reduction and dysfunction (Beausejour, Citation2007; Liu et al., Citation2012; Morel et al., Citation2013). Specifically, oxidized low-density lipoprotein (ox-LDL), remnants of lipoprotein in hypercholesterolemia, high glucose in type II diabetes, and angiotensin II in hypertension can accelerate the senescence of EPCs (Chen et al., Citation2007; Imanishi et al., Citation2004; Zhou et al., Citation2010). Interestingly, the substances mentioned above are also risk factors for cardiovascular and cerebrovascular diseases. In contrast, many cardiovascular drugs can prevent EPC senescence, including statins, angiotensin II receptor blockers, and peroxisome proliferator-activated receptor (PPAR) agonists (Imanishi et al., Citation2005, Citation2008a; Liu et al., Citation2012). Thus, the cell therapy based on EPCs may be a new strategy to treat ischemic cardiovascular and cerebrovascular diseases, and a new target for anti-ischemic drugs.
Angelica sinensis (Oliv.) Diels (Apiaceae) is a perennial herb distributed widely in China. It is reported that the dried roots of A. sinensis contain phthalides, polysaccharides, coumarin, and ferulate. Angelica sinensis has been used as a traditional Chinese medicine for the treatment of diverse diseases, including gynecological ailments, fatigue, anemia, and hypertension (Chen et al., Citation2013). Moreover, Danggui-Buxue-Tang, a medicinal formulae composed primarily of A. sinensis, has been certified as an anti-ischemic agent (Gao et al., Citation2005). As the primary water-soluble component, A. sinensis polysaccharides (ASP) may play a key role in activity of A. sinensis formulae. However, the cardiovascular and cerebrovascular activities of ASP have not been evaluated. Thus, in view of the importance of EPCs in cardiovascular and cerebrovascular diseases, we hypothesized that ASP may have anti-ischemic effects on EPCs. In this study, we investigated the protective effects of ASP against ox-LDL-induced senescence of EPCs and explored its underlying molecular mechanisms.
Materials and methods
Isolation of A. sinensis polysaccharide
The roots of A. sinensis were purchased from Minxian County of China on 2 September 2012, and they were identified by Prof. Rui Yang according to the Pharmacopeia of China. Voucher specimens of the plant were deposited at the School of Bioengineering, Xihua University, China. The dried roots were defatted with alcohol, and the ASPs were extracted with boiling distilled water. The extracts were vacuum concentrated and centrifuged at 3500 rpm for 10 min. The supernatant was collected, precipitated with alcohol, and centrifuged to obtain crude granular polysaccharides. The crude polysaccharides were dissolved and dialyzed in distilled water, followed by concentration. Proteins were removed by repeated freezing and thawing, and the samples were lyophilized to obtain the ASP. ASP was analyzed using the phenol–sulfuric acid method (Dubois et al., Citation1956). Briefly, ASP was hydrolyzed with 1 M sulfuric acid and converted to alditol acetates. The acetates were analyzed by gas chromatography (Agilent 7890A, Agilent Technologies, Inc., Wilmington, DE), equipped with a HP-5 capillary column (Agilent Technologies, Inc., Wilmington, DE) (30 m × 0.32 mm × 0.33 μm) and an Agilent 5975CMS detector (Agilent Technologies, Inc., Wilmington, DE).
Isolation, culture, differentiation, and characterization of BM-derived EPCs
All animal procedures were performed in compliance with the Experimental Animal Regulations approved by the National Science and Technology Commission of China (Protocol number: [1997]593). EPCs were harvested and cultured according to previously described methods (Lai & Liu, Citation2014). Briefly, the BM was isolated from the femur and tibia of 3 - to 4-week-old male Wistar rats (Sichuan Professional Committee on Experimental Animals, Chengdu, China). Mononuclear cells (MNCs) were isolated from rat BM by density-gradient centrifugation in Ficoll (density 1.083) and cultured on human fibronectin (FN)-coated (Sigma-Aldrich, St Louis, MO) culture dishes containing endothelial growth medium (EGM-2) supplemented with EGM-2-MV-SingleQuots (Clonetics, San Diego, CA) and 20% fetal bovine serum (FBS; Gibco, Gaithersburg, MD). Adherent cells were thoroughly washed on day 4 to remove unattached cells, and the fresh culture medium was added. The culture medium was changed every 3 d thereafter. The EPCs were rendered dormant by incubation in a serum-free medium for 24 h before treatment.
To identify EPCs, a fluorescence assay was performed using the adherent MNCs after culturing for 7 d. Fluorescent staining was used to detect the binding of fluorescein isothiocyanate (FITC)-labeled Ulex europaeus agglutinin (UEA-1; Sigma-Aldrich, St Louis, MO) and the uptake of 1,1′-dioctadecyl-3,3,3′,3′-tetramethyl-indocarbocyanine-labeled acetylated LDL (DiI-acLDL; Molecular Probe, NY). Cells were first incubated with DiI-acLDL (10 μg/mL) at 37°C for 4 h, and then fixed with 2% paraformaldehyde for 15 min. The cells were washed and then incubated with 10 μg/mL of UEA-1 for 1 h. After staining, the cells were observed through an inverted fluorescent microscope (Olympus, Tokyo, Japan). BM-EPCs were identified as attached cells that were double positive for both UEA-1 binding and DiI-acLDL uptake (Lefèvre et al., 2007). Immunofluorescent staining was performed on BM-EPCs to detect the expression of the vascular endothelial growth factor receptor 2 (VEGFR-2) and CD133, using antibodies against VEGFR-2 (Zsbio, Beijing, China) and CD133 (Zsbio, Beijing, China), respectively.
Preparation of ox-LDL
ox-LDL was prepared according to a method described previously (Lai & Liu, Citation2014). Briefly, native LDL (nLDL; Sigma-Aldrich, St. Louis, MO) was incubated with CuSO4 (10 μM) at 37 °C for 24 h. The culture was dialyzed against a sterile solution of NaCl (150 mM), ethylenediamine tetraacetic acid (EDTA; 1 mM), and polymixin B (100 μg/mL; pH 7.4) overnight. The availability of ox-LDL was verified by the production of thiobarbituric acid and agarose gel electrophoresis.
Treatment of EPCs
After culturing for 7 d, the cells were digested into cell suspension using 0.25% (w/v) trypsin. An equal number of cells were seeded on 24-well plates and divided into four groups: control, incubated with vehicle (EGM-2); Model, incubated with ox-LDL (10 μg/mL, 24 h); ASP-high, ox-LDL (10 μg/mL, 24 h) plus ASP (100 μg/mL, 48 h); and ASP-low, ox-LDL (10 μg/mL, 24 h) plus ASP (20 μg/mL, 48 h). In addition, to confirm the role of the phosphoinositide 3-kinase/protein kinase B (PI3K/Akt) pathway in this process, the ASP-high group (Wort.) was prepared, consisting of EPCs pre-treated with Wortmannin (100 nM, 45 min; Sigma-Aldrich, St. Louis, MO), a specific inhibitor of the PI3K/Akt signalling pathway.
Senescence-associated β-galactosidase (SA-β-gal) staining
As a biomarker for cellular senescence, senescence-associated β-galactosidase (SA-β-gal) activity was measured using the Senescence Detection kit (Beyotime, Haimen, China) according to the protocol of the manufacturer. In brief, quiescent EPCs grown to 70% confluence on a 6-well plate were exposed to the different experimental conditions. The cells were washed in phosphate-buffered saline (PBS), fixed for 3 min at room temperature in 2% paraformaldehyde, washed, and incubated for 16 h at 37°C (without CO2) with fresh SA-β-gal stain solution. The cells were photographed, and the percentage of SA-β-gal positive perinuclear blue cells was determined by counting five random fields.
Telomerase activity assay
EPCs grown to 70% confluence were lysed with CHAPS buffer. The lysates were centrifuged at 15 000g for 30 min and protein concentrations of the supernatant were measured using Bradford assay. Telomerase activity was determined using the TeloTAGGG Telomerase PCR ELISA Plus Kit (Roche, Mannheim, Germany), the TRAP-PCR reaction mixture contained 0.5 μg protein of each sample, 10 pM of each primer (TS: 5′-AATCCGTCGAGCAGAGTTAGGGTTAG-3′; CX: 5′-CCCTTACCCTTACCCTTACCCTAA-3′), 10 mM dNTPs, 1 U Taq DNA polymerase, and 1 × Taq PCR buffer. The real-time PCR parameters were as follows: 1 cycle at 30°C, 30 min; 1 cycle at 95°C, 2 min; and 45 cycles (94°C, 15 s; 59°C 60 s; 45°C 10 s).
Superoxide anion detection
Superoxide anion production in EPCs was assessed by staining with the fluorescent dye dihydroethidium (DHE) as described previously (Thum et al., Citation2007). DHE displays blue fluorescence in the unoxidized form, whereas it displays red fluorescence when oxidized to 2-hydroxyethidium by the superoxide anion. Therefore, DHE staining is an indirect measure of the production of reactive oxygen species (ROS). Briefly, the EPCs (1 × 105 cells/mL) treated with the different experimental conditions were incubated with 10 µM DHE (Beyotime, Haimen, China) at 37°C for 30 min. After washing with PBS twice, the fluorescence intensity (488 nm) of the different groups was immediately analyzed with the FV1000 (Olympus, Tokyo, Japan).
Western blot analysis
EPCs were lysed in a Triton X-100-based lysis buffer (10% Triton X-100, 10% glycerol, 150 mM NaCl, 20 mM Tris [pH 7.5], 2 mM EDTA, 1 mM phenylmethanesulfonyl fluoride [PMSF], 10 μg/mL aprotinin, and 10 μg/mL leupeptin) for 1 h, and the nuclear and the cellular debris were cleared by centrifugation. Proteins were separated using sodium dodecyl sulfate polyacrylamide gel electrophoresis (SDS-PAGE) and blotted onto nitrocellulose membranes (Bio-Rad, Hercules, CA). The membranes were blocked with skim milk in Tris-buffered saline (10 mM Tris, 100 mM NaCl, pH 7.5) containing 0.05% Tween 20 for 2 h. After washing the membrane with Tris-buffered saline, primary antibodies, including anti-human telomerase reverse transcriptase (hTERT; 1:1000), anti-phospho-hTERT-Ser1125 (1:500), anti-Akt (1:1000), anti-phospho-Akt-Ser473 (1:500), and anti-gp91-phox (1:500) (Santa Cruz, Dallas, TX), were added and incubated overnight at 4°C. After being thoroughly washed, the membranes were incubated with horseradish peroxidase (HRP)-conjugated immunoglobulin G (IgG; 1:40 000, Boster, Wuhan, China) for 1 h at room temperature. Blots were developed using enhanced chemiluminescence, and the quantification was performed.
Statistical analysis
All data are shown as the mean ± standard deviation (SD). Data were analyzed by one-way ANOVA, followed by a Dunnett’s test. A p value less than 0.05 was considered statistically significant.
Results
Characterization of polysaccharides
ASP was a kind of amorphous powder and had a carbohydrate content of 97%. From the chemical analysis, the component monosaccharides of ASP, determined using by GC, were arabinose, glucose, rhamnose, galactose, and galacturonic acid with a molar ratio of 10.4:4.7:1.0:5.2:8.0.
Characterization of BM-derived EPCs
MNCs harvested from the BM of Wistar rats and cultured for 7 d developed a spindle-shaped appearance (i.e., morphology of early EPCs). Most adherent cells (≥ 90%) took up DiI-acLDL and bound UEA-1 after culture for 7 d. EPCs were identified as attached cells that were double positive for DiI-acLDL uptake and UEA-1 binding (). The results of the immunofluorescent staining indicated that most of the cells were double positive for CD133 and VEGFR-2 (). These cells were confirmed to be EPCs.
Figure 1. Characterization of bone marrow-derived endothelial progenitor cells (EPCs). (A) Green fluorescence (upper left) represents cells positive for fluorescein isothiocyanate-labeled Ulex europaeus agglutinin (FITC-UEA-l). Red fluorescence (upper middle) represents cells positive for 1,1′-dioctadecyl-3,3,3′,3′-tetramethylindocarbocyanine-labeled acetylated low-density lipoprotein (DiI-acLDL). Yellow fluorescence (upper right) represents differentiated endothelial progenitor cell-like adherent cells that were double-positive for uptake of DiI-acLDL and binding with FITC-UEA-l. (B) The cultured mononuclear cells (MNCs) were further characterized by immunofluorescent staining using the EPC-specific markers, CD133 (middle left) and vascular endothelial growth factor receptor 2 (VEGFR-2, middle right), and the nuclear marker 4,6-diamidino-2-phenylindole (DAPI, lower left). The cells further differentiated to cobblestone-like late EPCs. Bars in figure = 50 μm.
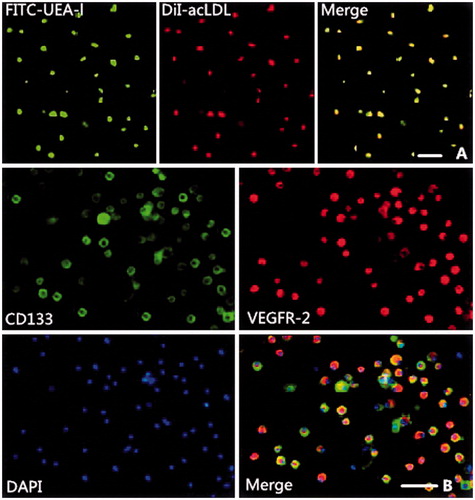
ASP prevented EPCs senescence
Senescent EPCs display some common features, including autophagic vacuoles and increased acidic β-galactosidase activity and lysosomal mass (Thijssen et al., Citation2009). To investigate whether ASP inhibited EPC senescence induced by ox-LDL, we measured the activity of senescence-associated acidic β-galactosidase. As shown in , after incubation with ox-LDL (10 μg/mL) for 24 h, the number of SA-β-Gal-positive cells increased significantly (model: 47.3 ± 12.2% versus control: 11.5 ± 4.6%; p < 0.05). ASP inhibited the senescence-like phenotype, including SA-β-gal activity and morphological changes (the percentage of SA-β-gal positive cells: ASP-high: 16.7 ± 8.1% versus model; p < 0.05). Moreover, the inhibitive effect was dose dependent, although the difference between the ASP-low and model groups was not statistically significant (ASP-low: 40.9 ± 15.0% versus model; p > 0.05). However, when EPCs were pre-treated with Wortmannin, the protective effect of ASP on EPC senescence was partially attenuated (Wort.: 29.8 ± 5.4% versus ASP-high; p < 0.05).
Figure 2. EPC senescence was evaluated by acidic-β-Gal activity. Representative photo-micrographs show SA-β-gal-positive cells (blue) in EPCs (A). The protective effects of ASP on the senescence of EPCs. Ox-LDL (model) increased senescence of EPCs compared with the control. Treatment of EPCs with 100 μg/mL ASP significantly decreased senescent EPCs. The beneficial effect of ASP was abrogated by Wortmannin partially. The lower histogram (B) showed changed percentage of SA-β-gal-positive cells after different treatments. Data are shown as mean ± SD (five random fields). §p < 0.05 versus control; *p < 0.05 versus model; #p < 0.05 versus ASP-high. The Bar = 50 μm.
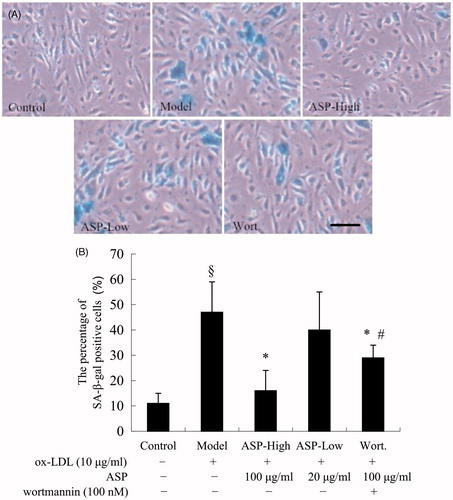
ASP decreased superoxide anion generation in EPCs
To investigate whether ASP inhibited ox-LDL-induced superoxide anion generation in EPCs, a superoxide (O2 − ) detection assay was performed using the oxidant-sensitive fluorescent probe DHE. As shown in , the fluorescence intensity was significantly higher in the model group than in the control group, whereas high doses of ASP markedly attenuated the fluorescence intensity after ox-LDL treatment. These results indicated that ox-LDL increased intracellular oxidative stress and that ASP attenuated this effect. As shown in , treatment of EPCs with ox-LDL (10 μg/mL) for 24 h significantly increased intracellular superoxide anion generation (model: 375 ± 32% versus control: 100%; p < 0.05). ASP dose dependently reduced superoxide generation induced by ox-LDL (ASP-high: 148 ± 38% versus model; p < 0.05).
Figure 3. Intracellular superoxide anion generation was determined by quantitative fluorescence imaging using DHE. (A) DHE was oxidized by superoxide anion, and then the oxidized product, 2-hydroxy-ethidium (HEt), inserted DNA and emitted red fluorescence. Thus, the fluorescence intensity was a marker of levels of intracellular superoxide anion. (B) Corresponding with the photomicrographs, representative histogram showing ox-LDL (model) significantly increased the production of superoxide anion in EPCs. Treatment of EPCs with 100 μg/mL ASP significantly decreased intracellular superoxide anion generation. Data are shown as mean ± SD (n = 5). §p < 0.05 versus control; *p < 0.05 versus model. The Bar = 40 μm.
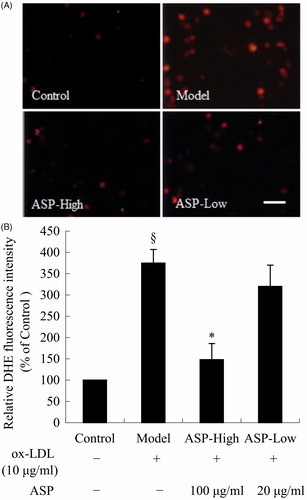
ASP induced telomerase activity
Telomere shortening and dysfunction are associated with cell senescence. Telomerase elongates telomeres and counteracts decrease of telomere length (Shuai et al., Citation2012). Thus, we measured telomerase activity using the telomeric repeat-amplification protocol (TRAP) assay. As shown in , ox-LDL decreased telomerase activity (model: 42 ± 9% versus control, 100%; p < 0.05), whereas the observed decrease in telomerase activity was dose dependently attenuated by ASP (ASP-high: 88 ± 7% and ASP-low: 60 ± 10% versus model; p < 0.05). However, Wortmannin markedly counteracted the effect of ASP on telomerase activity (Wort.: 66 ± 16% versus ASP-high; p < 0.05).
Figure 4. A histogram illustrating the results of telomeric repeat-amplification protocol (TRAP) assay. Ox-LDL significantly diminished telomerase activity, and this effect was partially abolished by co-treatment with ASP. To examine whether the PI3K-Akt cascade is involved in the effect of ASP, EPCs were pre-treated with Wortmannin (100 nM), a selective PI3K inhibitor. Interestingly, the beneficial effect of ASP was abrogated by Wortmannin partially. Data are shown as mean ± SD (five random fields). §p < 0.05 versus control; *p < 0.05 versus model; #p < 0.05 versus ASP-high.
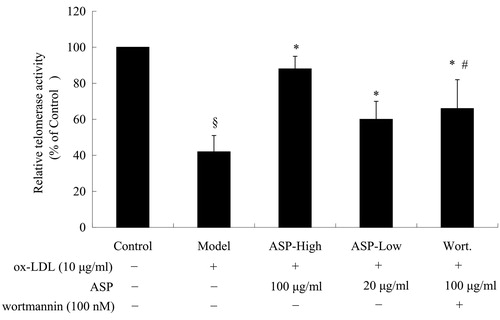
ASP induced telomerase activity via the Akt/hTERT and NADPH oxidase pathway
To further explore the molecular mechanisms involved in the anti-senescence activity of ASP, the expression level of key telomerase-related proteins was assessed by western blotting. Immunoblotting was performed with a specific phospho-Akt (p-Akt) antibody-targeting serine 473 phosphorylation and a phospho-hTERT (p-hTERT) antibody-targeting serine 1125 phosphorylation. As shown in , the total Akt and hTERT levels remained unchanged. Thus, we set the protein expression ratio of p-Akt/Akt or p-hTERT/hTERT of the control group to 100%. Ox-LDL decreased Akt (model: 59 ± 7% versus control: 100%; p < 0.05) and hTERT (model: 41 ± 12% versus control: 100%; p < 0.05) phosphorylation. ASP dose dependently reversed the reduction of p-Akt expression (ASP-high: 136 ± 18% and ASP-low: 88 ± 15%, versus model; p < 0.05). Similarly, the inhibition of hTERT phosphorylation was also attenuated by ASP (ASP-high: 90 ± 9% and ASP-low: 58 ± 19% versus model; p < 0.05). Nevertheless, treatment with Wortmannin reversed the effect of ASP on Akt and hTERT phosphorylation (p-Akt: Wort.: 72 ± 20% versus ASP-high; p-hTERT: Wort.: 44 ± 6% versus ASP-high; p < 0.05). Additionally, we investigated the influence of ASP on the NADPH oxidase pathway. Incubation of EPCs with ox-LDL upregulated the expression of gp91-phox, a subunit of NADPH oxidase (model: 407 ± 45% versus control: 100%; p < 0.05); however, this increase was dose dependently attenuated by ASP (ASP-high: 130 ± 10% and ASP-low: 228 ± 69% versus model; p < 0.05).
Figure 5. Effect of ASP on protein kinase B (Akt) phosphorylation at Serine 473 and human telomerase reverse transcriptase (hTERT) phosphorylation at serine 1125. Western blot analysis was performed on EPCs that were treated with ox-LDL, ox-LDL plus ASP, or ox-LDL plus ASP plus Wortmannin. (A) Ratio of p-Akt/total Akt in EPCs lysates; (B) ratio of p-hTERT/total hTERT in EPCs lysates; (C) expression of gp91-phox. Densities of the p-Akt (p-hTERT or gp91-phox) bands were corrected with respect to total Akt (total hTERT or β-actin) and then the p-Akt/total Akt or p-hTERT/total hTERT expression ratio was calculated. The ratio of the control group was assigned a value of 1.0 for statistical analysis. In short, ox-LDL decreased phosphorylation of Akt and hTERT. Treatment with ASP inhibited the ox-LDL-induced reduction of p-Akt and p-hTERT; however, Wortmannin pre-treatment suppressed p-Akt and p-hTERT expression in ASP-treated EPCs. In NADPH oxidase pathway, ox-LDL markedly increased expression of gp91-phox, a subunit of NADPH. The results caused elevation of superoxide anion level in EPCs. Data are shown as mean ± SD (five random fields). §p < 0.05 versus control; *p < 0.05 versus model; #p < 0.05 versus ASP-high.
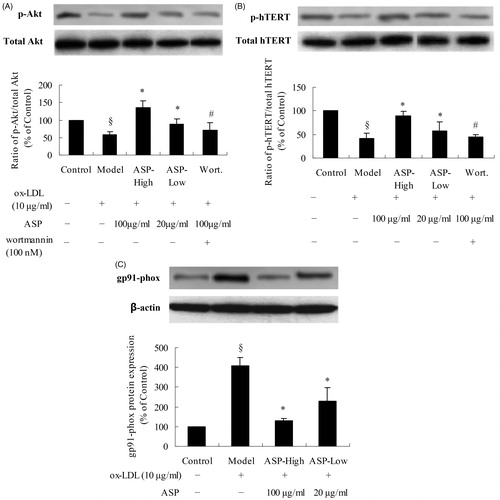
Discussion
Cellular senescence is the irreversible growth arrest of individual mitotic cells, leading to a radically altered phenotype that is thought to impair tissue function and predispose tissues to disease development and/or progression, such as atherosclerosis (Burton, Citation2009). Telomere shortening is a pivotal molecular event during cellular senescence. A telomere is a region of repetitive nucleotide sequences (TTAGGG) at each end of a chromatid, which protects the end of the chromosome from deterioration or from fusion with neighboring chromosomes. Over time, due to cell division, the telomere ends become shorter. There is accumulating evidence that progressive telomere shortening is closely related to cardiovascular disease (Minamino et al., Citation2008). To proliferate beyond the senescent checkpoint, cells must restore telomere length. This can be achieved by telomerase, an enzyme with reverse-transcriptase activity. This enzyme is absent in differentiated somatic cells; however, telomerase reactivation has been detected in most cancer cells, stem, and progenitor cells. The introduction of telomerase was proposed as a method to combat aging via cell therapy and a possible method to regenerate tissue (Natesan, Citation2005; Shawi et al., Citation2008). As a progenitor cell, EPCs show telomerase activity. However, inhibition of telomerase activity accelerates the senescence of EPCs in some pathological states, including hyperglycemia, hyperlipidemia, and oxidative stress (Imanishi et al., Citation2008b; Yang et al., Citation2011).
Telomerase activity is regulated by many factors (Nicholls et al., Citation2011). ROS, generated by NADPH oxidase, and phosphorylation of hTERT, a subunit of human telomerase and a downstream effector of the PI3K/Akt pathway, can influence the activity of telomerase (Paneni et al., Citation2013; Takano et al., Citation2008). In the present study, we showed that ASP inhibited EPC senescence induced by ox-LDL and revealed that NADPH oxidase and the Akt pathway mediated this effect.
In this study, we showed, for the first time, that ASP inhibited the onset of ox-LDL-mediated senescence in EPCs, which was associated with a decrease in SA-β-gal positive cells and a recovery of telomerase activity. These findings partially explained the anti-ischemic application of A. sinensis preparations (Gao et al., Citation2005). In this study, ox-LDL, a pro-atherosclerotic factor, accelerated the onset of EPC senescence, whereas ASP relieved this effect.
The mechanism by which ASP inhibits the onset of cellular senescence seemed to involve telomerase activity. Our data showed that ASP dose dependently enhances telomerase activity, indicating that ASP could inhibit EPC senescence by inducing telomerase activity. However, the molecular mechanism by which ASP-enhanced telomerase activity remains unclear. In the present study, we have shown that ASP significantly increased phosphorylation of Akt and hTERT. Recent studies have suggested that the serine–threonine kinase Akt increases telomerase activity through phosphorylation of hTERT in cancer cells (Shi et al., Citation2014). As a pivotal subunit of telomerase, the activity of hTERT was modulated by Akt. ASP decreased cell senescence, whereas the AKT pathway inhibitor Wortmannin partially abolished the protective effects of ASP, suggesting that activation of the Akt/hTERT pathway plays a crucial role in the protective effects of ASP. Beside activation of Akt/hTERT pathway, our results suggested that ASP dose dependently prevented the upregulation of gp91-phox induced by ox-LDL. As a subunit of NADPH oxidase, the expression level of gp91-phox reflected the activity of NADPH oxidase, which is a major source of ROS (Elnakish et al., Citation2013). The data from the superoxide (O2−) detection assay confirmed that ASP exerted an anti-oxidative effect in EPCs. As oxidative stress regulates telomerase activity, NADPH oxidase plays an important role in EPC senescence. Combined, ASP increased the phosphorylation of Akt, thereby promoting hTERT phosphorylation and telomerase activation. Further, ASP exerted an anti-oxidative effect and downregulated the expression of gp91-phox, which inhibited NADPH oxidase activity. The inhibition reduced intracellular superoxide ion levels and oxidative stress. Thus, ASP restored the activity of telomerase and attenuated senescence in EPCs by two mechanisms.
In the present study, we chose ox-LDL to induce EPC senescence, because it is a major risk factor for AS induced by hyperlipidaemia. Ox-LDL can impair vascular endothelium and endothelial repair. In our previous study, higher concentrations of ox-LDL (100 μg/mL) induced EPC apoptosis (Lai & Liu, Citation2014), whereas lower concentrations of ox-LDL (10 μg/mL) induced EPC senescence. Nevertheless, compounds which can improve EPC number or function in the presence of ox-LDL may have potential as therapeutics in cardiovascular and cerebrovascular diseases. Thus, targeting EPCs may become a new strategy for discovering and developing drugs from natural products.
Conclusion
The results of the present study demonstrate that treatment of EPCs with ASP remarkably attenuates the harmful effects of ox-LDL via augmentation of Akt/hTERT phosphorylation and inhibition of oxidative stress.
Declaration of interest
The authors report no conflicts of interest. The authors alone are responsible for the content and writing of this article.
References
- Asahara T, Murohara T, Sullivan A, et al. (1997). Isolation of putative progenitor endothelial cells for angiogenesis. Science 275:964–7
- Beausejour C. (2007). Bone marrow-derived cells: The influence of aging and cellular senescence. Handb Exp Pharmacol 180:67–88
- Burton DG. (2009). Cellular senescence, ageing and disease. Age 31:1–9
- Chen XP, Li W, Xiao XF, et al. (2013). Phytochemical and pharmacological studies on Radix Angelica sinensis. Chin J Nat Med 11:577–87
- Chen YH, Guh JY, Chuang TD, et al. (2007). High glucose decreases endothelial cell proliferation via the extracellular signal regulated kinase/p15(INK4b) pathway. Arch Biochem Biophys 465:164–71
- Dubois M, Gilles KA, Hamilton JK, et al. (1956). Colorimetric method for determination of sugars and related substances. Anal Chem 28:350–6
- Elnakish MT, Hassanain HH, Janssen PM, et al. (2013). Emerging role of oxidative stress in metabolic syndrome and cardiovascular diseases: Important role of Rac/NADPH oxidase. J Pathol 231:290–300
- Gao D, Song J, Hu J, et al. (2005). Angiogenesis promoting effects of Chinese herbal medicine for activating blood circulation to remove stasis on chick embryo chorio-allantoic membrane. Zhongguo Zhong Xi Yi Jie He Za Zhi 25:912–15
- Imanishi T, Hano T, Nishio I. (2005). Angiotensin II accelerates endothelial progenitor cell senescence through induction of oxidative stress. J Hypertens 23:97–104
- Imanishi T, Hano T, Sawamura T, Nishio I. (2004). Oxidized low-density lipoprotein induces endothelial progenitor cell senescence, leading to cellular dysfunction. Clin Exp Pharmacol Physiol 31:407–13
- Imanishi T, Kobayashi K, Kuroi A, et al. (2008a). Pioglitazone inhibits angiotensin II-induced senescence of endothelial progenitor cell. Hypertens Res 31:757–65
- Imanishi T, Tsujioka H, Akasaka T. (2008b). Endothelial progenitor cells dysfunction and senescence: Contribution to oxidative stress. Curr Cardiol Rev 4:275–86
- Krankel N, Luscher TF, Landmesser U. (2012). “Endothelial progenitor cells” as a therapeutic strategy in cardiovascular disease. Curr Vasc Pharmacol 10:107–24
- Lai P, Liu Y. (2014). Echinocystic acid, isolated from Gleditsia sinensis fruit, protects endothelial progenitor cells from damage caused by oxLDL via the Akt/eNOS pathway. Life Sci 114:62–9
- Lavoie JR, Stewart DJ. (2012). Genetically modified endothelial progenitor cells in the therapy of cardiovascular disease and pulmonary hypertension. Curr Vasc Pharmacol 10:289–99
- Lefèvre J, Michaud SÉ, Haddad P, et al. (2007). Moderate consumption of red wine (cabernet sauvignon) improves ischemia-induced neovascularization in ApoE-deficient mice: Effect on endothelial progenitor cells and nitric oxide. FASEB J 21:3845–52
- Libby P, Ridker PM, Hansson GK. (2011). Progress and challenges in translating the biology of atherosclerosis. Nature 473:317–25
- Liu Y, Wei J, Hu S, Hu L. (2012). Beneficial effects of statins on endothelial progenitor cells. Am J Med Sci 344:220–6
- Minamino T, Komuro I. (2008). Role of telomeres in vascular senescence. Front Biosci 13:2971–9
- Morel O, Jesel L, Abbas M, Morel N. (2013). Prothrombotic changes in diabetes mellitus. Semin Thromb Hemost 39:477–88
- Natesan S. (2005). Telomerase extends a helping hand to progenitor cells. Trends Biotechnol 23:1–3
- Nicholls C, Li H, Wang JQ, Liu JP. (2011). Molecular regulation of telomerase activity in aging. Protein Cell 2:726–38
- Paneni F, Osto E, Costantino S, et al. (2013). Deletion of the activated protein-1 transcription factor JunD induces oxidative stress and accelerates age-related endothelial dysfunction. Circulation 127:1229–40, e1–21
- Raval Z, Losordo DW. (2013). Cell therapy of peripheral arterial disease: From experimental findings to clinical trials. Circ Res 112:1288–302
- Ruan C, Shen Y, Chen R, et al. (2013). Endothelial progenitor cells and atherosclerosis. Front Biosci (Landmark Ed) 18:1194–201
- Shawi M, Autexier C. (2008). Telomerase, senescence and ageing. Mech Ageing Dev 129:3–10
- Shi YA, Zhao Q, Zhang LH, et al. (2014). Knockdown of hTERT by siRNA inhibits cervical cancer cell growth in vitro and in vivo. Int J Oncol 45:1216–24
- Shuai L, Li X, He Q, et al. (2012). Angiogenic effect of endothelial progenitor cells transfected with telomerase reverse transcriptase on peritubular microvessel in five out of six subtotal nephrectomy rats. Ren Fail 34:1270–80
- Takano H, Murasawa S, Asahara T. (2008). Functional and gene expression analysis of hTERT overexpressed endothelial cells. Biologics 2:547–54
- Thijssen DH, Torella D, Hopman MT, Ellison GM. (2009). The role of endothelial progenitor and cardiac stem cells in the cardiovascular adaptations to age and exercise. Front Biosci (Landmark Ed) 14:4685–702
- Thum T, Fraccarollo D, Thum S, et al. (2007). Differential effects of organic nitrates on endothelial progenitor cells are determined by oxidative stress. Arterioscler Thromb Vasc Biol 27:748–54
- Yang DG, Liu L, Zhou SH, et al. (2011). Remnant-like lipoproteins may accelerate endothelial progenitor cells senescence through inhibiting telomerase activity via the reactive oxygen species-dependent pathway. Can J Cardiol 27:628–34
- Zhou Z, Hu CP, Wang CJ, et al. (2010). Calcitonin gene-related peptide inhibits angiotensin II-induced endothelial progenitor cells senescence through up-regulation of klotho expression. Atherosclerosis 213:92–101