Abstract
Hepatitis C virus nonstructural protein 3 (NS3) helicase is a promising target for developing new therapeutics. In this study, we identified cholesterol sulfate (CS) as a novel NS3 helicase inhibitor (IC50 = 1.7 ± 0.2 µM with a Hill coefficient of 3.9) by screening the extracts from marine organisms. The lack of the sulfate group, sterol structure or alkyl side chain of CS diminished the inhibition, suggesting that an anion binding and hydrophobic region in NS3 may be a target site of CS. It was further found that CS partly inhibits NS3–RNA binding activity, but exerted no or less inhibition against ATPase and serine protease activities. Moreover, we demonstrated that CS probably does not bind to RNA. Our findings suggest that CS may inhibit NS3 helicase not by abolishing the other NS3 activities but by inducing conformational changes via interaction with possible allosteric sites of NS3.
Introduction
More than 170 million people are chronically infected worldwide with the hepatitis C virus (HCV), a major etiological agent responsible for the development of chronic hepatitis, liver cirrhosis and hepatocellular carcinomaCitation1. The current standard therapy is mainly based on the combined use of pegylated interferon-alfa and ribavirin; however, the treatment has severe side effects and is the least effective in about 50% of patients chronically infected with HCV genotype 1Citation2 and about 20% of those infected with HCV genotypes 2 and 3Citation3. It is therefore urgently needed to develop direct-acting antivirals that target the viral proteins involved in HCV replication as a promising therapeutic strategyCitation4.
HCV nonstructural protein 3 (NS3) helicase is one possible target for new drug development because of its essential role in viral replicationCitation5,Citation6. HCV is a single-positive-stranded RNA virus belonging to the genus Hepacivirus of the Flaviviridae family. The viral genome contains a single open reading frame encoding a polyprotein that is processed by virus-encoded and host cellular proteases into structural and nonstructural proteins. One nonstructural protein is the protease/helicase NS3, a multifunctional enzyme with serine protease and NTPase/helicase at the N- and C-termini, respectivelyCitation7. NS3 helicase can unwind double-stranded RNA (dsRNA), double-stranded DNA and RNA/DNA heteroduplexes in a 3′–5′ direction using any nucleoside triphosphate as the energy sourceCitation8–11. Although the exact role of NS3 helicase in the viral life cycle is still unclear, a fully functioning NS3 helicase is essential for HCV RNA replicationCitation5, making NS3 helicase a potential drug target. The NS3 helicase inhibitors have already been found to act on NS3 helicase mainly through the inhibition of ATPase and nucleic acid bindingCitation12. However, none of the inhibitors have yet been tested in clinical trials, probably because their mechanism of action is not yet entirely elucidated, hampering the rational design of such inhibitors. Thus, there is still a high potential for finding novel candidates of NS3 helicase inhibitors.
Current drug discovery largely depends on the high-throughput screening of synthetic chemical librariesCitation13. Nevertheless, the majority of new drugs are still generated from either natural products or analogs based on natural products in almost all therapeutic areasCitation14,Citation15. Natural products are potential sources of structurally diverse chemical compounds with various biological activities. Among natural sources, marine organisms harbor highly diverse microorganisms that are major sources of secondary metabolites with unique chemical structures. Indeed, since the 1970s, more than 15 000 structurally diverse natural products with different bioactivities have been discovered from marine microorganisms, algae and invertebratesCitation16. Therefore, it is reasonable to expect marine organisms as potential chemical sources of starting materials for driving new drug development.
In this study, we carried out screening of NS3 helicase inhibitors in the extracts from marine organisms by fluorescence helicase assay based on photoinduced electron transfer (PET) as described in our previous studyCitation17. In the course of purification, cholesterol sulfate (CS), which was isolated from the extract of a crinoid, was identified as a novel NS3 helicase inhibitor with an IC50 in the low micromolar range. We examined the inhibitory activity of the compounds lacking the sulfate group, sterol structure or alkyl side chain of CS to speculate on the functional groups or moieties of CS necessary for the inhibition of NS3 helicase. The inhibitory mechanism of CS was further examined in relation to the inhibitory activity of CS against the other NS3 activities (ATPase, RNA binding and serine protease activities) that are linked with NS3 helicase.
Materials and methods
Preparation of extracts from marine organisms
Specimens of marine organisms were collected by scuba diving in Okinawa, Japan, and kept frozen before use. The specimens were chopped into small pieces and soaked in acetone for 20 h and then in methanol for 6 h. The acetone and methanol solutions were combined and concentrated, and the residual materials were partitioned into the ethyl acetate and aqueous layers. Each layer was dried to obtain residues.
Screening for HCV NS3 helicase inhibitors
The fluorescence helicase assay based on PET was performed as described in our previous studyCitation17 with modifications of the reaction mixture composition. The dsRNA substrate was prepared by annealing the 5′-BODIPY FL-labeled fluorescence strand (5′-CUAUUACCUCCACCCUCAUAACCUUUUUUUUUUUUUU-3′) to the quencher strand (5′-GGUUAUGAGGGUGGAGGUAAUAG-3′) at a 1:2 molar ratio. The dsRNA substrate has the 3′-overhang that is necessary for NS3 helicase to bind RNA prior to the duplex unwinding. The capture strand (5′-CTATTACCTCCACCCTCATAACC-3′), which is complementary to the quencher strand, prevents the unwound duplexes from reannealing. None of the above three strands is self-complementary. The fluorescence strand was purchased from J-Bio 21 Corporation (Tokyo, Japan), which was labeled with BODIPY FL at the 5′-end via an aminohexylphosphate linker with a six-carbon spacer. The quencher and capture strands were purchased from Japan Bio Services (Saitama, Japan). The reaction mixture contained 25 mM MOPS-NaOH (pH 6.5), 3 mM MgCl2, 2 mM dithiothreitol, 4 U of RNasin (Promega, WI), 50 nM dsRNA substrate, 100 nM capture strand, 5 mM ATP, an extract from a marine organism and 240 nM NS3 in a total volume of 20 µL. Each extract from a marine organism diluted with DMSO was added to the reaction mixture at a final concentration in the range of 17.5–32.5 µg/mL. The full-length HCV NS3 protein with serine protease and NTPase/helicase was expressed and purified as described previouslyCitation17.
The reaction was started by adding HCV NS3 helicase and performed at 37 °C for 30 min using a LightCycler 1.5 (Roche Diagnostics, Basel, Switzerland). Fluorescence intensity was recorded every 5 s from 0 to 5 min, and then every 30 s from 5 to 30 min. Helicase activity was calculated as the initial reaction velocity relative to that of the control without a sample but with DMSO. IC50 was calculated using KaleidaGraph (Synergy Software, Reading, PA) by fitting plots of % activity versus [I] using Equation (1)Citation18:
where h is the Hill coefficient and [I] is the inhibitor concentration.
RNA unwinding assay
NS3 RNA helicase assay was performed as described previouslyCitation7,Citation19 with some modifications. In brief, the substrate for annealing two complementary RNA oligonucleotides, 5′-AGAGAGAGAGGUUGAGAGAGAGAGAGUUUGAGAGAGAGAG-3′ (40-mer, template strand) and 5′-CAAACUCUCUCUCUCUCAACAAAAAA-3′ (26-mer, release strand), was purchased from Shanghai GenePharma (Shanghai, China). The release strand was labeled at the 5′-end with [γ-32P] ATP (Muromachi Yakuhin, Tokyo, Japan) using T4 polynucleotide kinase (Toyobo, Osaka, Japan) at 37 °C for 60 min and purified by phenol-chloroform extraction. The template and the labeled release strands were annealed at a molar ratio of 3:1 (template/release), denatured at 80 °C for 5 min, and slowly renatured at 23 °C for 30 min in an annealing buffer that contained 20 mM Tris-HCl (pH 8.0), 0.5 M NaCl and 1 mM EDTA. The partial duplex RNA substrate was purified on a G-50 microcolumn (GE Healthcare, WI) and stored at −20 °C in H2O containing 0.25 U of RNasin Plus (Promega, WI) per µL.
The assay of NS3 RNA helicase inhibition by CS was conducted in 20 µL of helicase reaction mixture that contained 25 mM MOPS-NaOH (pH 7.0), 2.5 mM DTT, 2.5 U of RNasin Plus (Promega, WI), 100 µg of BSA per mL, 3 mM MgCl2, 300 nM NS3 protein and 0.4 nM 32P-labeled partial duplex RNA substrate with increasing concentrations of CS (as indicated) and preincubated at 23 °C for 15 min. After adding 5 mM ATP, the reaction was carried out at 37 °C for 30 min and stopped by adding 5 µL of helicase termination buffer that contained 0.1 M Tris (pH 7.5), 20 mM EDTA, 0.5% SDS, 0.1% Nonidet P-40, 0.1% bromophenol blue, 0.1% xylene cyanol and 25% glycerol. The inhibition of NS3 helicase was analyzed on a 10% native Tris/borate/EDTA (TBE) polyacrylamide gel, and the labeled RNAs were visualized using an FLA-9000 image reader (Fujifilm, Tokyo, Japan). Similar to the PET assay, IC50 was calculated using KaleidaGraph (Synergy Software, PA) by fitting plots of % activity versus [I] using Equation (1).
Fluorescent intercalator displacement assay
The intercalatory property of CS was studied by fluorescent intercalator displacement assay as described previouslyCitation20 with some modifications. The dsRNA used as a substrate of NS3 helicase in the PET assay was incubated for 1 h at room temperature with CS at the indicated concentrations in 25 mM MOPS-NaOH (pH 6.5), and then subjected to electrophoresis in 20% acrylamide gel in 1 × TBE. The gel was subsequently stained with ethidium bromide. Neomycin was used as a positive control that potentially intercalates into dsRNACitation21. The compounds that intercalate into dsRNA inhibit ethidium bromide intercalation and the dsRNA band disappears partially or totally.
Chemicals
CS and six chemical compounds with structurally similar properties to CS were obtained commercially. CS, cholesterol and dehydroepiandrosterone sulfate were purchased from Avanti Polar Lipids (AL). Bis-(sodium sulfopropyl)-disulfide, di-n-butyl sulfate, sodium dodecyl sulfate and sodium sulfate were purchased from Wako (Osaka, Japan). For the assays, the compounds were dissolved in DMSO.
ATPase assay
NS3 ATPase activity was directly determined by monitoring [γ-32P] ATP hydrolysis by thin-layer chromatography as described in previous reportsCitation7,Citation19 with slight modifications. The reaction mixture contained 25 mM MOPS-NaOH (pH 7.0), 1 mM dithiothreitol, 5 mM MgCl2, 5 mM CaCl2, 1 mM [γ-32P] ATP (Muromachi Yakuhin, Tokyo, Japan), 300 nM NS3, 0.1 µg/µL poly (U) single-stranded RNA (ssRNA) (Sigma-Aldrich, MO) and increasing concentrations of CS (as indicated) in a volume of 10 µL. The reaction was conducted at 37 °C for 10 min and stopped by adding 10 mM EDTA. After the reaction, each reaction mixture was spotted at 2 µL onto a polyethyleneimine cellulose sheet (Merck, Darmstadt, Germany) and developed in 0.75 M LiCl/1 M formic acid solution for 20 min. The cellulose sheet was dried, and the released [γ-32P] phosphoric acid was visualized using an Image Reader FLA-9000 and quantified using Multi Gauge software V 3.11 (Fujifilm, Tokyo, Japan). ATPase activity was calculated as the ratio of the signal intensity derived from the released Pi in the sample with CS to that in the control sample without the inhibitor but with DMSO.
RNA binding assay
NS3–RNA binding activity was determined by gel mobility shift assay as previously describedCitation19,Citation22. The ssRNA (5′-UGAGGUAGUAGGUUGUAUAGU-3′) synthesized by Gene Design (Osaka, Japan) was labeled at the 5′-end with [γ-32P] ATP (Muromachi Yakuhin, Tokyo, Japan) using T4 polynucleotide kinase (Toyobo, Osaka, Japan) at 37 °C for 60 min and purified by the phenol-chloroform extraction method. The reaction mixture contained 30 mM Tris-HCl (pH 7.5), 100 mM NaCl, 2 mM MgCl2, 1 mM dithiothreitol, 20 U of RNasin plus (Promega, WI), 300 nM NS3, 5 fmol of 32P-labeled ssRNA and increasing concentrations of CS (as indicated) in a volume of 20 µL. The reaction was performed at room temperature for 15 min. An equal volume of a dye solution containing 0.025% bromophenol blue and 10% glycerol in 0.5× TBE was added to each reaction mixture and the mixture was loaded onto a 6% native-polyacrylamide gel. The labeled RNA bands were visualized using an Image Reader FLA-9000 and quantified using Multi Gauge Software V 3.11 (Fujifilm, Tokyo, Japan). RNA binding activity was calculated as the ratio of the signal intensity derived from the NS3-ssRNA complex in the sample with CS to that in the control sample without the inhibitor but with DMSO.
Serine protease assay
The fluorescence NS3 serine protease assay based on fluorescence resonance energy transfer was conducted using reagents provided in a SensoLyte™ 520 HCV protease assay kit (AnaSpec, CA). In brief, the NS3 protein with a twofold excess of the NS4A cofactor peptide (Pep4AK) was prepared in 1× assay buffer provided in the kit. HCV NS3/4A protease was mixed with increasing concentrations of CS and incubated at 37 °C for 15 min. The reaction was started by adding the 5-FAM/QXL 520 substrate in 20 µL of the total reaction mixture that contains 180 nM HCV NS3/4 A protease and increasing concentrations of CS (as indicated), and performed at 37 °C for 120 min using a LightCycler 1.5 (Roche Diagnostics, Basel, Switzerland). Fluorescence intensity was recorded every minute up to 120 min. NS3 serine protease activity was calculated as the initial reaction velocity in the sample with CS relative to that in the control sample without the inhibitor but with DMSO.
Results
Screening for HCV NS3 helicase inhibitors in extracts from marine organisms
To obtain novel NS3 helicase inhibitors, the extracts from marine organisms were screened by fluorescence helicase assay based on PET. We examined 41 extracts prepared from marine organisms, three of which decreased NS3 helicase activity to less than 30%, suggesting that these extracts contain potential NS3 helicase inhibitors. For the isolation of NS3 helicase inhibitors, we purified the ethyl acetate extract from a crinoid (NN-9-2-1) that exerted the most significant inhibition among the three extracts.
In the course of purification (Supplementary Table S1), we obtained three fractions from NN-9-2-1 that inhibited NS3 helicase to less than 50% (NN-9-8-1, NN-9-8-3 and NN-9-8-4). The inhibition by NN-9-8-4 was most potent; thus, the fraction was further purified into three fractions (NN-9-30-1, NN-9-30-2 and NN-9-30-3). Consequently, the major component of NN-9-47-2, which was isolated from NN-9-30-2, was identified as sodium CS () from its NMR spectra (Supplementary Figure S1), negative ESIMS m/z 465.30575 [M − H]− and ICPMS (Na) data. As shown in Supplementary Figure S2, the IC50 of NN-9-47-2 was 0.8 µg/mL. The purity of CS in NN-9-47-2 was calculated as less than or comparable to 89% from the 1H NMR spectra (Supplementary Figure S1A). The concentration of CS at the IC50 of NN-9-47-2 (0.8 µg/mL) was estimated to be about 0.7 µg/mL (1.4 µM). We further examined a commercially available CS (Avanti Polar Lipids, AL), and its IC50 was 1.7 ± 0.2 µM (), which is similar to the estimated IC50 of CS in NN-9-47-2, indicating that CS is the NS3 helicase inhibitor in NN-9-47-2. The observed Hill coefficient was 3.9 (). As the Hill coefficient was greater than unity, the data suggest that complete inhibition of NS3 helicase requires more than one CS binding event so that the stoichiometry of the interaction is greater than one-to-one. All the subsequent biochemical kinetic analyses were performed using the commercially available CS.
Figure 2. Dose–response curve for the inhibition of NS3 helicase by CS. NS3 helicase activity was measured by PET assay. NS3 helicase activity of the sample with CS was calculated relative to that of the control sample without the inhibitor but with DMSO. The data are presented as the mean ± standard deviation for three replicates. The solid and dashed lines indicate the curve fittings in which h = 3.9 and 1, respectively.
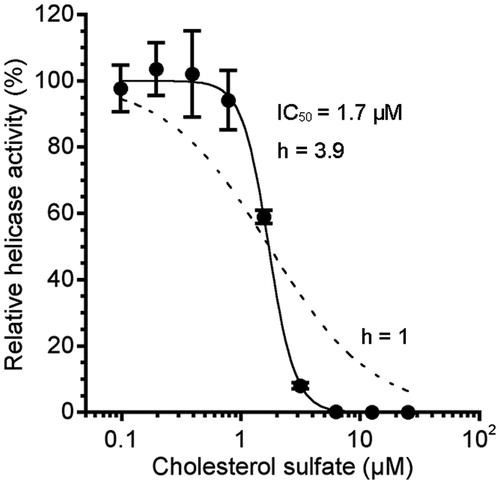
Inhibition of NS3 helicase-catalyzed RNA unwinding by CS
A series of control experiments were carried out to confirm the inhibition of NS3 helicase by CS, because of the possibility that CS interferes with PET assay by causing apparent fluorescence intensity changes with possible turbidity and fluorescence quenching, or by forming nucleic acid adducts that either block the helicase or prevent the capture strand from annealing to the reaction products.
The effect of CS on turbidity and fluorescence quenching in PET assay was examined. There was no effect of CS at 50 µM on the absorbance at a wavelength of approximately 500 nm, which is relevant to the excitation/emission maxima of BODIPY FL (505/513 nm) in PET assay (Supplementary Figure S3). In addition, CS did not decrease fluorescence intensity in PET assay at 50 µM in the absence of NS3 helicase (Supplementary Figure S4). Our results indicate that there was no effect of CS on turbidity and fluorescence quenching in PET assay. Furthermore, we performed RNA helicase assay using radioisotope-labeled RNA () and demonstrated the inhibition of NS3 helicase by CS with the IC50 of 1.6 µM, which is similar to that obtained by PET assay (1.7 µM). The helicase activity was abolished at CS concentrations >10 µM, which is consistent with the inhibition at >7 µM CS in PET assay (). The Hill coefficient was determined as more than unity (1.8), suggesting that more than one CS binds to one molecule of NS3 helicase.
Figure 3. Inhibition of NS3 helicase by CS. (A) Autoradiography of RNA unwinding assay with 32P-labeled RNA. The heat-denatured single-strand RNA (26-mer) and the partial duplex RNA substrate were applied to lanes 1 and 2, respectively. The duplex RNA was reacted with NS3 (300 nM) in the presence of CS (lanes 3–7, 0–20 μM). The resulting samples were subjected to native polyacrylamide gel electrophoresis. (B) Graphical representation of the experiment shown in (A). NS3 helicase activity was calculated as the ratio of signal intensity derived from the ssRNA in the sample with CS to that of the control sample without the inhibitor but with DMSO. The solid and dashed lines indicate the curve fittings in which h = 1.8 and 1, respectively.
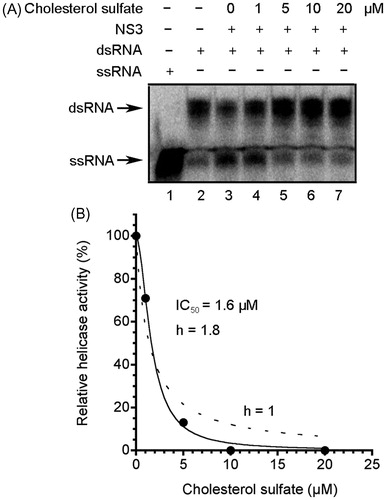
The intercalatory property of CS into RNA was examined by fluorescent intercalator displacement assayCitation20. It was suggested that CS has no or low ability to intercalate into RNA at concentrations up to 500 µM (Supplementary Figure S5), and this is probably due to its negatively charged sulfate group that potentially prevents CS from binding into nucleic acids that are also negatively charged. For the same reason, it is less likely that CS has the ability to bind to either the major or minor grooves of nucleic acids; therefore, we considered that CS probably does not form nucleic acid adducts.
We further examined the inhibition by the six chemical compounds that possess structurally similar properties to CS, cholesterol and five sulfate compounds, by PET assay ( and ). Cholesterol, which lacks the sulfate group of CS, did not inhibit NS3 helicase at 50 µM, suggesting that the sulfate group is necessary for the inhibition. Four sulfate compounds that lack the sterol structure of CS, namely, bis-(sodium sulfopropyl)-disulfide, di-n-butyl sulfate, sodium dodecyl sulfate and sodium sulfate, exerted no inhibition of NS3 helicase at 50 µM. Moreover, dehydroepiandrosterone sulfate, which lacks only the alkyl side chain of CS, exerted less inhibition at 50 µM. These findings suggest that the sulfate group and the hydrophobicity of the sterol structure with the alkyl side chain of CS are necessary for the inhibition of NS3 helicase, and an anion binding and hydrophobic region in NS3 may be a target site of CS.
Table 1. Effects of CS and the structurally related compounds on NS3 helicase activity.*
Effect of CS on NS3 ATPase activity
As the unwinding ability of NS3 helicase is dependent on ATP hydrolysis, the amount of inorganic phosphate (Pi) released from radioisotope-labeled ATP was measured to determine the effect of CS on the ATPase activity of NS3. The released Pi was separated by thin-layer chromatography, and then visualized by autoradiography. The density of the upper spots corresponding to Pi, which represents ATPase activity, increased dose-dependently up to ∼200% at CS concentrations ranging from 1 to 10 µM (). However, ATPase activity gradually decreased at CS concentrations >10 µM and reached about 50% at 100 µM CS (data not shown). Because the IC50 of CS for NS3 helicase was 1.7 µM and the helicase activity was abolished at CS concentrations >7 µM (), the inhibition of ATPase seems less relevant to that of NS3 helicase; thus, CS is considered not to inhibit NS3 helicase through the inhibition of ATPase.
Figure 5. Effect of CS on NS3 ATPase activity. (A) Autoradiography of ATPase assay with [γ-32P] ATP. Lane 1 contains the control reaction mixture in the absence of NS3. Lanes 2–5 show the ATP hydrolysis reaction at increasing concentrations of CS with poly (U) ssRNA. (B) Graphical representation of the experiment shown in (A). ATPase activity was calculated as the ratio of the signal intensity derived from the released Pi in the sample with CS to that in the control sample without the inhibitor but with DMSO. The data are presented as the mean ± standard deviation for two replicates.
![Figure 5. Effect of CS on NS3 ATPase activity. (A) Autoradiography of ATPase assay with [γ-32P] ATP. Lane 1 contains the control reaction mixture in the absence of NS3. Lanes 2–5 show the ATP hydrolysis reaction at increasing concentrations of CS with poly (U) ssRNA. (B) Graphical representation of the experiment shown in (A). ATPase activity was calculated as the ratio of the signal intensity derived from the released Pi in the sample with CS to that in the control sample without the inhibitor but with DMSO. The data are presented as the mean ± standard deviation for two replicates.](/cms/asset/3125ed1d-54ef-4fdc-b1d4-bae412c19df7/ienz_a_766607_f0005_b.jpg)
Effect of CS on NS3–RNA binding activity
Because nucleic acid binding is required for NS3 helicase activity, the effect of CS on NS3–RNA binding activity was examined by gel mobility shift assay (). Lane 2 is the control lane for examining the nonspecific binding of ssRNA to bovine serum albumin. The density of the upper bands corresponding to the NS3-ssRNA complex, which represents NS3–RNA binding activity, increased to ∼120% at 0.8 µM CS, followed by a decrease at CS concentrations of 0.8–5 µM (). Taken together with the results seen in , NS3 helicase and RNA binding activities are likely to decrease at a similar CS concentration range. This result indicates that inhibition of NS3 helicase is associated with a decrease in the NS3–RNA binding activity. However, the RNA binding activity was not completely abolished even at higher CS concentrations up to 20 µM (data not shown), in which range NS3 helicase activity was completely abolished. These data suggest that the inhibition of NS3–RNA binding is partially involved in that of NS3 helicase.
Figure 6. Effect of CS on NS3 RNA binding activity. (A) Autoradiography of gel mobility shift assay with 32P-labled ssRNA. Lanes 1 and 2 contain the control reaction mixtures with heat-denatured ssRNA alone or 300 nM bovine serum albumin (BSA) instead of NS3, respectively. Lanes 3–9 show the binding of NS3 helicase to ssRNA at increasing concentrations of CS. (B) Graphical representation of the experiment shown in (A). RNA binding activity was calculated as the ratio of signal intensity derived from the NS3–ssRNA complex in the sample with CS to that of the control sample without the inhibitor but with DMSO. The data are presented as the mean ± standard deviation for two replicates.
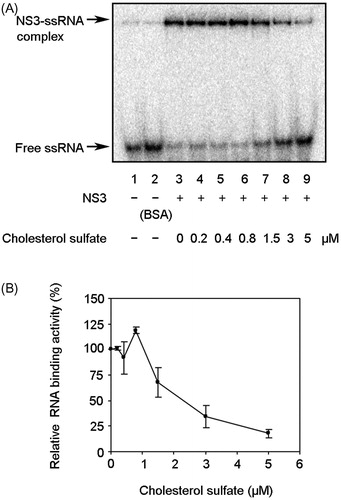
Effect of CS on NS3 serine protease activity
It has been reported that NS3 helicase activity is interdependently linked to NS3 serine protease activityCitation23–25. We examined the effect of CS on NS3 serine protease activity by fluorescence serine protease activity assay. The fluorescence time courses at increasing CS concentrations (0–100 µM) were examined. The serine protease activity slightly decreased, and the inhibition of serine protease was at most ∼20% at 20 and 100 µM CS (). Moreover, the activities at higher CS concentrations of 250 and 500 µM were 99 and 95%, respectively (data not shown), indicating that serine protease activity does not decrease in a dose-dependent manner but rather it fluctuates. The result also indicates that CS does not inhibit NS3 helicase through the inhibition of serine protease because the IC50 of CS for NS3 helicase was 1.7 µM, which seems less relevant to the inhibition of serine protease. Unlike the RI assay results on ATPase and RNA binding activities, serine protease activity did not increase over 100% of the control at low CS concentrations of 1–10 µM.
Discussion
HCV NS3 helicase has been considered as one possible target for new drug development owing to its essential activity for viral replication. In this study, we carried out screening of NS3 helicase inhibitors in the extracts from marine organisms that are potential chemical sources with structurally diverse natural compounds. Through the screening assay and a series of purification steps for the extracts from marine organisms, CS was identified as a potential inhibitor of HCV NS3 helicase from the extract from a crinoid. CS is a component of cell membranes where it has a stabilizing role and is abundant in the human body with many physiological characteristicsCitation26. Although CS is already known to inhibit various human proteasesCitation27–30 and DNase ICitation31, to the best of our knowledge, CS has not been reported as an inhibitor of a viral protein.
Small-molecule helicase inhibitors are considered to act through the inhibition of NTP binding, nucleic acid binding, NTP hydrolysis or NDP release, coupling of NTP hydrolysis to translocation and unwinding of nucleic acid, or unwinding by sterically blocking helicase translocationCitation6. In addition, owing to the interdependent linkage between NS3 helicase and NS3 serine protease activitiesCitation23–25, the inhibition of NS3 serine protease may also lead to the inhibition of NS3 helicase; furthermore, the compounds that intercalate into the strands of double-stranded nucleic acids could also inhibit NS3 helicaseCitation12.
This study demonstrated that CS partially inhibits NS3–RNA binding activity, but exerted no or less inhibition against ATPase and serine protease activities. In addition, we showed by fluorescent intercalator displacement assay that CS probably does not bind to RNA. Our findings suggest that CS is less likely to inhibit NS3 helicase by abolishing the other NS3 activities linked with NS3 helicase (ATPase, RNA binding and serine protease activities). Taken together with the Hill coefficients, which were determined as greater than unity from two different assays ( and ), it may also be suggested that multiple CSs prevent NS3 helicase from unwinding dsRNA by interacting with possible allosteric sites of NS3. NS3 helicase is a multidomain protein that consists of domains 1, 2 and 3. Among them, domain 2 mainly functions in ATP-dependent unwinding by the dynamic switching motion between the other domainsCitation32. Domains 1 and 3 hold the 3′-end of the nucleic acid chain when domain 2 moves forward, whereas domain 2 holds the 5′-end of the nucleic acid chain when domains 1 and 3 move forwardCitation32. It may be suggested that CS inhibits the retention between the domains and the nucleic acid chain by the allosteric conformational changes of the enzyme during the movements of the domains with the enzyme still partly bound to the nucleic acid chain. This inhibition model could be depicted as the “slipping” or “idling” of the enzyme-inhibitor complex, preventing NS3 from translocating on the nucleic acid chain.
Another inhibitory mechanism of CS might be that CS stimulates RNA binding activity to prevent NS3 helicase from translocating and being released from dsRNA. However, our data suggest that the stimulation of RNA binding is less relevant to the inhibition of NS3 helicase, because RNA binding activity decreased to ∼70% at 1.5 µM (), which is almost identical to the IC50; moreover, RNA stimulation was limited to a narrow CS concentration range (∼1 µM).
ATPase stimulation was also observed at the same concentrations at which the activation of RNA binding occurred. As NS3 ATPase activity is dependent on the NS3–RNA binding activityCitation33, it may be suggested that the activation of ATPase is due to the activation of RNA binding. However, ATPase activation at higher CS concentrations is probably not relevant to the activation of RNA binding, which is clearly abolished at those CS concentrations. Because NS3 couples ATPase activity to helicase activity by utilizing the free energy derived from ATP hydrolysis for either translocation or unwinding reaction, it is considered that the activation of ATPase should lead to that of NS3 helicase. However, such activation of NS3 helicase in concordance with ATPase activation was not observed. This lack of NS3 helicase activation might be due to the inhibition of the process that couples ATPase activity to helicase activity. Future studies will be needed to clarify this inhibitory mechanism of CS.
Conclusions
This study demonstrated that CS isolated from crinoid inhibits NS3 helicase (IC50 = 1.7 ± 0.2 µM) with partial inhibition of NS3–RNA binding activity and no or less inhibition against ATPase and serine protease activities. As CS is considered to inhibit NS3 helicase probably not by the inhibition of ATPase but through the interaction with possible allosteric sites of NS3, CS may less likely inhibit other similar ATPase enzymes, providing potentially useful information on future drug design for the novel NS3 helicase inhibitors that are expected to be more specific and less toxic. Furthermore, because CS is a component of cell membranes, both CS and HCV NS3 helicase may coexist inside the cell and could possibly have some interactions with each other. Future in vivo studies on CS might lead to the development of advanced drug design strategies for the novel NS3 helicase inhibitors.
Declaration of interest
The authors report no conflicts of interest. The authors alone are responsible for the content and writing of this article.
Acknowledgements
The authors thank Dr S. Nishikawa (AIST) for his kind gift of the expression plasmid pT7/His-NS3 containing the N-terminal His-tagged full-length HCV NS3 and Mr N. Nagahama (University of the Ryukyus) for his technical assistance.
References
- Ciesek S, Manns MP. Hepatitis in 2010: the dawn of a new era in HCV therapy. Nat Rev Gastroenterol Hepatol 2011;8:69–71
- Fried MW, Shiffman ML, Reddy KR, et al. Peginterferon alfa-2a plus ribavirin for chronic hepatitis C virus infection. N Engl J Med 2002;347:975–82
- Manns MP, McHutchison JG, Gordon SC, et al. Peginterferon alfa-2b plus ribavirin compared with interferon alfa-2b plus ribavirin for initial treatment of chronic hepatitis C: a randomised trial. Lancet 2001;358:958–65
- Opar A. Excitement grows for potential revolution in hepatitis C virus treatment. Nat Rev Drug Discov 2010;9:501–3
- Lam AM, Frick DN. Hepatitis C virus subgenomic replicon requires an active NS3 RNA helicase. J Virol 2006;80:404–11
- Kwong AD, Rao BG, Jeang KT. Viral and cellular RNA helicases as antiviral targets. Nat Rev Drug Discov 2005;4:845–53
- Gallinari P, Brennan D, Nardi C, et al. Multiple enzymatic activities associated with recombinant NS3 protein of hepatitis C virus. J Virol 1998;72:6758–69
- Kim DW, Gwack Y, Han JH, Choe J. C-terminal domain of the hepatitis C virus NS3 protein contains an RNA helicase activity. Biochem Biophys Res Commun 1995;215:160–6
- Tai CL, Chi WK, Chen DS, Hwang LH. The helicase activity associated with hepatitis C virus nonstructural protein 3 (NS3). J Virol 1996;70:8477–84
- Gwack Y, Kim DW, Han JH, Choe J. Characterization of RNA binding activity and RNA helicase activity of the hepatitis C virus NS3 protein. Biochem Biophys Res Commun 1996;225:654–9
- Gwack Y, Kim DW, Han JH, Choe J. DNA helicase activity of the hepatitis C virus nonstructural protein 3. Eur J Biochem 1997;250:47–54
- Belon CA, Frick DN. Helicase inhibitors as specifically targeted antiviral therapy for hepatitis C. Future Virol 2009;4:277–93
- Macarron R, Banks MN, Bojanic D, et al. Impact of high-throughput screening in biomedical research. Nat Rev Drug Discov 2011;10:188–95
- Newman DJ, Cragg GM. Natural products as sources of new drugs over the last 25 years. J Nat Prod 2007;70:461–77
- Li JW, Vederas JC. Drug discovery and natural products: end of an era or an endless frontier? Science 2009;325:161–5
- Salomon CE, Magarvey NA, Sherman DH. Merging the potential of microbial genetics with biological and chemical diversity: an even brighter future for marine natural product drug discovery. Nat Prod Rep 2004;21:105–21
- Tani H, Akimitsu N, Fujita O, et al. High-throughput screening assay of hepatitis C virus helicase inhibitors using fluorescence-quenching phenomenon. Biochem Biophys Res Commun 2009;379:1054–9
- Copeland RA. Evaluation of enzyme inhibitors in drug discovery: a guide for medicinal chemists and pharmacologists. New York: Wiley; 2005:111–40
- Salam KA, Furuta A, Noda N, et al. Inhibition of hepatitis C virus NS3 helicase by manoalide. J Nat Prod 2012;75:650–4
- Stankiewicz-Drogon A, Palchykovska LG, Kostina VG, et al. New acridone-4-carboxylic acid derivatives as potential inhibitors of hepatitis C virus infection. Bioorg Med Chem 2008;16:8846–52
- Mikkelsen NE, Johansson K, Virtanen A, Kirsebom LA. Aminoglycoside binding displaces a divalent metal ion in a tRNA-neomycin B complex. Nat Struct Biol 2001;8:510–14
- Huang Y, Liu ZR. The ATPase, RNA unwinding, and RNA binding activities of recombinant p68 RNA helicase. J Biol Chem 2002;277:12810–15
- Frick DN, Ginzburg O, Lam AM. A method to simultaneously monitor hepatitis C virus NS3 helicase and protease activities. Methods Mol Biol 2010;587:223–33
- Dahl G, Sandström A, Akerblom E, Danielson UH. Effects on protease inhibition by modifying of helicase residues in hepatitis C virus nonstructural protein 3. FEBS J 2007;274:5979–86
- Frick DN. The hepatitis C virus NS3 protein: a model RNA helicase and potential drug target. Curr Issues Mol Biol 2007;9:1–20
- Strott CA, Higashi Y. Cholesterol sulfate in human physiology: what’s it all about? J Lipid Res 2003;44:1268–78
- Iwamori M, Iwamori Y, Ito N. Sulfated lipids as inhibitors of pancreatic trypsin and chymotrypsin in epithelium of the mammalian digestive tract. Biochem Biophys Res Commun 1997;237:262–5
- Iwamori M, Iwamori Y, Ito N. Regulation of the activities of thrombin and plasmin by cholesterol sulfate as a physiological inhibitor in human plasma. J Biochem 1999;125:594–601
- Sato J, Denda M, Nakanishi J, et al. Cholesterol sulfate inhibits proteases that are involved in desquamation of stratum corneum. J Invest Dermatol 1998;111:189–93
- Ito N, Iwamori Y, Hanaoka K, Iwamori M. Inhibition of pancreatic elastase by sulfated lipids in the intestinal mucosa. J Biochem 1998;123:107–14
- Iwamori M, Suzuki H, Kimura T, Iwamori Y. Shedding of sulfated lipids into gastric fluid and inhibition of pancreatic DNase I by cholesterol sulfate in concert with bile acids. Biochim Biophys Acta 2000;1487:268–74
- Raney KD, Sharma SD, Moustafa IM, Cameron CE. Hepatitis C virus non-structural protein 3 (HCV NS3): a multifunctional antiviral target. J Biol Chem 2010;285:22725–31
- Suzich JA, Tamura JK, Palmer-Hill F, et al. Hepatitis C virus NS3 protein polynucleotide-stimulated nucleoside triphosphatase and comparison with the related pestivirus and flavivirus enzymes. J Virol 1993;67:6152–8