Abstract
This study sought to explore the mechanism of anti-inflammatory effect of triphala in lipopolysaccharide (LPS)-stimulated RAW 264.7 macrophages and in adjuvant-induced arthritic rats. In stimulated RAW 264.7 cells, triphala (100–300 μg/ml) significantly suppressed production of inflammatory mediators (e.g. TNFα, IL-1β, IL-6, MCP-1, VEGF, NO, PGE2), intracellular free radicals and release of lysosomal enzymes (e.g. acid phosphatase, β-galactosidase, N-acetyl glucosamindase and cathepsin D) in a dose-related manner. With triphala, mRNA levels of genes for pro-inflammatory TNFα, IL-1β, IL-6 and MCP-1, inflammatory iNOS and COX-2 enzymes and NF-κBp65 were down-regulated in the stimulated cells; in contrast, there was up-regulation of heme oxygenase-1 (HO-1) expression. Western blot analyses revealed that triphala suppressed the protein expression of NF-κB p65 and p-NF-κB p65 in the stimulated cells, which subsequently reduced over-expression of TNFα, IL-17, iNOS and COX-2 in a manner similar to that observed with BAY 11-7082, an IκB kinase inhibitor. Immunofluorescence analysis revealed inhibition of p-NF-κB p65 nuclear translocation and COX-2 protein expression caused by triphala. Consistent with these findings, the animal studies presented confirmed that triphala exhibited anti-inflammatory effects in a rat adjuvant-induced arthritis model by reducing of inflammatory mediator (e.g. IL-17, COX-2 and RANKL) expression via inhibition of NF-κB activation. Taken together, the results here demonstrated that triphala has potential anti-inflammatory applications that could be used for the treatment of inflammatory disorders, including rheumatoid arthritis.
Introduction
Inflammation is a complex and multiple process produced in response to different stimuli such as infection, chemical exposure, tissue damage, trauma or lipopolysaccharide endotoxin (LPS) (Choudhari et al. Citation2013). Macrophages are major inflammatory cells that play vital roles in immune response, allergy and inflammation. Activation by foreign stimuli like LPS activate the transcription factor nuclear factor (NF)-κB and mitogen-activated protein kinases (MAPKs). These events lead to the cellular release of inflammatory mediators (such as nitric oxide [NO] and prostaglandin (PG)- E2), pro-inflammatory cytokines like tumor necrosis factor (TNF)-α, interleukin (IL)-1β and IL-6, hydrolytic enzymes (e.g. acid phosphatase, cathepsin-D and β-glucouronidase), reactive oxygen species (ROS) and inflammatory enzymes such as inducible nitric oxide synthase (iNOS) and cyclooxygenase-2 (COX-2) (Biswas & Lewis Citation2010; Grivennikov et al. Citation2010).
The generation of these pro-inflammatory mediators is mainly regulated through the NF-κB and MAPK pathways (Li et al. Citation2012). On the other hand, activation of the NF-κB and MAPK pathways may also be achieved by elevated levels of free radicals and inflammatory cytokines. Heme oxygenase (HO-1), an essential enzyme in heme catabolism, has been reported to have an anti-inflammatory property by suppressing the production of pro-inflammatory cytokines during oxidative stress (Ryter et al. Citation2002).
Excessive production of the inflammatory mediators and enzymes by activated immune cells like macrophages play a vital role in the pathophysiology of many inflammatory diseases like rheumatoid arthritis and atherosclerosis. It has been speculated that inhibition of the formation of these inflammatory mediators and/or of the NF-κB signaling pathway in association with an up-regulation of HO-1 could serve as a useful therapeutic approach to treat many inflammation-based pathologies. This goal has provided one basis underlying the development of potential anti-inflammatory drugs.
Triphala is a commonly used herbal formulation derived from fruits of three trees, e.g. Indian gooseberry (Emblica officinalis Gaertn, family Euphobiaceae), Belleric myrobalan (Terminalia belerica Linn, family Combretaceae) and Chebulic myrobalan (Terminalia chebula Retzr, Combretaceae) (Sandhyaa et al. Citation2006). This formulation has been prescribed for treatment of a wide variety of conditions like infection, obesity, anemia, fatigue, constipation and against infectious diseases, including tuberculosis, pneumonia and AIDS (El-Mekkawy et al., Citation1995). Triphala has been shown to be a rich source of anti-oxidants including Vitamin C, ellagic, gallic and chebulinic acids, bellericanin, β-sitosterol and flavanoids (Jagetia et al. Citation2002).
Previous investigations have shown that triphala imparted several biological effects, including acting like an anti-oxidant (Takagi & Sanashiro Citation1996), anti-tumor (Kaur et al. Citation2005), anti-diabetic (Sabu & Kuttan 2002), anti-proliferative, anti-mutagenic (Kaur et al. Citation2002) and radio-protective (Jagetia et al. Citation2002) agent. Our own preliminary studies with triphala have shown it also imparts anti-inflammatory, immunomodulatory and lysosomal membrane-stabilizing effects in arthritic animal models (Rasool & Sabina Citation2007; Sabina et al. Citation2009; Kalaiselvan & Rasool Citation2015a,Citationb). Nevertheless, there remains no information on potential mechanisms underlying the anti-inflammatory action of triphala on inflammatory cascades and/or cell signaling pathways.
The RAW 264.7 macrophage cell line is a well-established model used to investigate how triphala extract might interfere with inflammation in macrophages (in vitro) induced by bacterial LPS (Wang et al. Citation2011). Adjuvant-induced arthritis, in an animal model, imitates the human pathophysiological condition, including chronic swelling in multiple joints due to accumulation of inflammatory cells, joint cartilage erosion, bone destruction; as such, it it routinely used to explore the effects of potent anti-inflammatory/anti-arthritic agents. As such, the purpose of the present work was to identify possible mechanisms of anti-inflammatory activity of triphala in LPS stimulated RAW 264.7 macrophages and in adjuvant-induced arthritic rats. It was hoped that the results of the present study could provide a molecular basis for the use of triphala against inflammatory diseases including rheumatoid arthritis.
Materials and methods
Chemicals
Dulbecco’s modified Eagle’s medium (DMEM), fetal bovine serum (FBS), penicillin and streptomycin were obtained from Life Technologies Inc. (Grand Island, NY). MTT (4,5-dimethylthiazol-2-yl)-2,5-diphenyltetrazolium bromide), DMSO (dimethyl sulfoxide), LPS (Type 0111:B4 from Escherichia coli), indomethacin (Indo) and TRIzol were purchased from Sigma (St. Louis, MO). High capacity cDNA reverse transcription and QuantiTect SYBR Green PCR kits were purchased, respectively, from Qiagen (Valencia, CA) and Applied Biosystems (Foster City, CA), for use in real time-PCR experiments. Monoclonal antibodies (MAb) against NF-κB p65, p-NF-κB p65, TNFα, iNOS, IL-17, RANKL and COX-2 were purchased from Cell Signaling Technology (Beverly, MA). Secondary horseradish peroxidase (HRP)-conjugated antibody was also purchased from Cell Signaling Technology. All other chemicals used here were purchased from HiMedia laboratories (Mumbai, India)
Preparation of aqueous extract of triphala
Commercially-available triphala powder (a mixture [equal proportions, 1:1:1 w/w/w] of dried/powdered fruits from Terminalia chebula, Emblica officinalis and Terminalia belerica) was obtained from the Indian Medical Practitioners Co-operative Stores and Society (IMCOPS, Chennai, India). A weighed amount of triphala powder was extracted for 24 h with double-distilled water in a flask, at room temperature, on a shaker. The extraction procedure was repeated until the water became colorless. All undissolved materials were removed by filtration through Whatman No. 1 filter paper. The resulting filtrate was lyophilized down to a dry powder and weighed (≈40% [w/w] yield). Portions of the extract were then suspended in distilled water to yield a stock solution of 1000 μg/ml for use in the experiments herein.
HPLC analysis of triphala
The presence of major active phyto-components in the lyophilized aqueous extract of triphala were assessed using a Prominence HPLC system (Shimadzu, Tokyo, Japan) equipped with a quaternary pump, autosampler, rheodyne-7725i injector, column oven and UV-Visible detector; all generated data were analyzed by associated LC real-time software. The separation of phytocomponents was carried out on a Shim Pack C-18 column (250 mm × 4.6 mm, id) packed with 5-μm particles. The optimized chromatographic conditions were: mobile phase consisting of gradients of solvents such as solvent A (phosphoric acid:water, 0.5:99.5 v/v) and solvent B (acetonitrile: acetic acid, 99:1 v/v), flow-rate of 1.5 ml/min and a column maintained at 25 °C. All phyto-components were detected in the UV range, i.e. 268 nm for tannic acid, 270 nm for gallic acid, 272 nm for ascorbic acid and 277 nm for chebulagic/chebulic acid. The presence of five expected major active components in the aqueous extract were identified by comparing with retention times (Rt) and UV absorption spectrum of their respective standards (Sigma).
In vitro studies
Cell culture
RAW 264.7 murine macrophages were purchased from the National Centre for Cell Sciences (Pune, India) and maintained in DMEM supplemented with 10% FBS, 100 U penicillin/ml and 10 mg streptomycin/ml in a humidified atmosphere of 5% CO2 at 37 °C. Cells were allowed to grow to 90–95% confluence, at which point they were washed with phosphate-buffered saline (PBS, pH 7.4) Thereafter, the cells were gently scraped from the flasks, pelleted and then used in the experiments outlined below. Unless elsewise specifically indicated, in each study, RAW 264.7 cells (1.5 × 106 cells/well) were cultured in 6-well plates and then treated with triphala (100–300 μg/ml) or Indo (20 μM) for 24 h and then with 1 μg LPS/ml for a further 24 h.
MTT assay for cell viability
RAW 264.7 macrophages were seeded in 96-well plates at 5 × 104 cells/well. The cells then received medium containing various concentrations of test extract (100, 200, 300, 400 or 500 μg extract/ml). As data on in vitro studies with triphala are limited in the literature, this dose range was selected to encompass one used in earlier studies by Kaur et al. (Citation2005) using another type of (i.e. acetone) extract with a variety of transformed cells. Based on this range, it was expected that a maximum non-cytotoxic drug concentration (and consequently a range to be used to establish any dose-responsivity of effects) could be defied for use in subsequent treatments herein.
After incubation for 24 h at 37 °C, the cells then received 1 μg LPS/ml and were incubated for a further 24 h. Parallel sets of cells received only medium in place of LPS. After this period, the medium was decanted, 20 μl MTT dye solution (5 mg/ml in PBS) was added to each well and the plate was incubated for 4 h at 37 °C. DMSO (200 μl) was then added to each well and the plate incubated for 15 min with gentle shaking at 37 °C to dissolve formazan crystals that formed in the cells. Relative viability was calculated by determining the absorbance at 570 nm in a Biotek microplate reader (Winooski, VT). Untreated control cells were assigned a relative viability of 100%.
Lysosomal enzymes assay
RAW 264.7 cells were seeded in 24-well plates (at 50 000 cells/well) and treated with triphala or Indo for 24 h prior to stimulation with LPS for 24 h. Thereafter, the cells were washed with PBS and then lysed by addition of ice-cold lysis buffer (400 mM NaCl, 0.5% Triton-X, 50 mM Tris-HCl [pH 7.4]). Lysate from the macrophages was then prepared by centrifugation at 10 000 × g for 10 min at 4 °C. The resulting cell-free supernatant was recovered and an aliquot removed for analysis of protein content by the method of Lowry et al. (Citation1951); the remaining material was stored at −80 °C until used in analyses of lysosomal enzymes. Appropriate control experiments were performed to measure the release of enzymes by untreated cells.
Acid phosphatase was assayed by the method of King (Citation1965) using disodium phenyl phosphate as substrate. Enzyme activity was expressed as μmoles phenol liberated/min/mg protein. Activity of β-galactosidase was assessed by the method of Rosenblit et al. (Citation1974) using 4-nitrophenyl-N-acetyl galactopyranoside as substrate; activity was expressed as μmoles p-nitro-phenol liberated/h/mg protein. N-Acetyl glucosaminidase activity was assessed by the method of Maruhn (Citation1976) using 4-nitrophenyl-N-acetyl glucosaminide as substrate; activity was expressed as μmoles p-nitrophenol formed/h/mg protein. Cathepsin D activity was assayed by the method of Biber et al. (Citation1981) using hemoglobin as substrate; activity was expressed as μmoles tyrosine liberated/h/mg protein.
Nitric oxide (NO) assay
RAW 264.7 cells (1.5 × 104 cells/well) were cultured in 6-well plates in DMEM without phenol red and then treated with different concentrations of triphala (100–300 μg/ml) or Indo (20 μM) for 24 h before NO production was induced by addition of LPS (1 μg/ml) and incubation for 24 h. Nitrite in the culture medium was then measured as an index of NO production using a Griess reaction. In brief, conditioned medium (100 μl) from each well was recovered and mixed with an equal volume of Griess reagent (0.2% naphthylethylenediamine dihydrochloride [NEDD] and 2% sulphanilamide in 5% phosphoric acid). After incubation at 37 °C for 15 min, the mixture absorbance was measured at 540 nm in the microplate reader. Values were compared with those from sodium nitrite standards assessed in parallel and the nitrite concentration in the media of treated cells was calculated.
Prostaglandin E2 (PGE2) assay
PGE2 levels in the conditioned medium of RAW 264.7 cells treated with different concentrations of triphala or Indo for 24 h and then with LPS for 24 h were assessed using an ELISA kit (Cayman Chemical, Ann Arbor, MI), according to manufacturer instructions. Absorbance in each sample was measured at 450 nm in the microplate reader.
Enzyme-linked immunosorbant assays (ELISA)
Cultured media from RAW 264.7 cells treated with different concentrations of triphala or Indo for 24 h and then with 24 h were collected and stored at −75 °C until analyzed for levels of TNFα, IL-1β, IL-6, MCP-1 and VEGF using ELISA kits (Peprotech, Rocky Hill, NJ), according to manufacturer instructions. The level of sensitivity of the kits was 32 pg TNFα/ml, 63 pg IL-1β/ml, 62 pg IL-6/ml, 63 pg MCP-1/ml and 12 pg VEGF/ml.
Quantitative real-time reverse-transcription polymerase chain reactions
After the RAW 264.7 cells had been treated with triphala or Indo for 24 h and then LPS for 24 h, the cells were rinsed with PBS and total RNA from each group isolated using TRIzol, according to manufacturer instructions. The quality/quantity of isolated total RNA was verified using UV spectroscopy. Each RNA sample was measured at 260 nm and 280 nm and the corresponding 260/280 ratio was used to assess the RNA purity.
Quantitative RT-PCR (qRT-PCR) was performed to measure TNFα, IL-1β, IL-6, MCP-1, iNOS, COX-2, NF-κB p65 and HO-1. RNA (2 μg/sample) was reverse transcribed using a high capacity cDNA reverse transcription kit (Applied Biosystems) and mRNA expression subsequently amplified using a Quantitect SYBR PCR kit (Venlo, the Netherlands). Gene-specific primers were designed manually using NCBI/primer-BLAST tool software and purchased from Sigma (). Transcription levels of each product were assessed utilizing a Step One real-time thermal cycler with SYBR Green PCR Master Mix according to manufacturer’s instructions. Amplification was performed using the following cycling conditions: 94 °C for 15 s, 60 °C annealing for 30 s and a 72 °C extension for 30 s. The fold-change in gene expression levels of target genes was calculated by normalizing to β-actin values using the 2−ΔΔCt comparative cycle threshold method.
Table 1. Primer sequences used for quantitative real-time PCR analysis of RNA.
Immunofluorescence assays
RAW 264.7 cells (1.5 × 104/well) were cultured in 6-well plates and treated with triphala or Indo for 24 h and then with LPS for 24 h. The cells were then recovered by gentle scraping and transferred to permanox plastic chamber slides whereon they were then fixed with ice-cold 100% methanol for 10 min in a −20 °C freezer. The cells/slides were then washed with PBS and blocked with 3% (w/v) bovine serum albumin in PBS for 30 min. The samples were then incubated overnight at 4 °C with rabbit anti-p-NF-κB p65 and anti-COX-2 mAb (1:200 dilution in PBS containing 1% bovine serum albumin [BSA]). After three rinses with PBS (5 min each), the cells were then incubated coated with specific Alexa Fluor (AF)-488-conjugated goat anti-rabbit secondary antibody (1:200 dilution in PBS/1% BSA) for 2 h at room temperature. The slides were then washed with PBS and incubated for 10 min at room temperature with 4,6-diamidino-2-phenylindole (DAPI; Invitrogen, San Francisco, CA) containing mounting medium. The specimens were then coverslipped and examined in a blinded manner using a BX-43 fluorescent microscope (Olympus, Tokyo, Japan). Images were analyzed using Image Pro Plus Image analysis software (Media Cybernetics, Rockville, MD). For each analysis, an area of 100 μm/slide was evaluated and the results were expressed based on the fluorescence intensity within the given region/field of visualization. A minimum of three fields/slide was assessed for the detection of COX-2 and p-NF-κB p65 proteins, respectively.
Western blotting analysis
RAW 264.7 cells (1.5 × 106/well) were treated with the various concentrations of triphala or Indo for 24 h or BAY 11-7082 (10 μM) for 1 h prior to stimulation with LPS (1 μg/ml) for the next 24 h. Thereafter, cell lysate were prepared in ice-cold RIPA buffer (150 mM NaCl, 1% IGEPA CA-630, 0.5% sodium deoxycholate, 0.1% SDS, 50 mM Tris [pH 8]) containing protease inhibitors (Sigma). Protein content in lysates was measured using a Bradford protein assay kit (BioRad, Hercules, CA). For the analyses, lysates (30 μg protein/lane) were electrophoretically resolved over 12% sodium dodecyl sulfate-polyacrylamide gels. Proteins were then electrotransferred to polyvinylidene fluoride membranes (PVDF); the blots were then blocked overnight at 4 °C in a solution of 5% (w/v) bovine serum albumin in TBST (Tris-buffered saline containing 0.1% Tween-20). The membranes were then incubated overnight at 4 °C with the desired primary polyclonal antibody individually against NF-κB p65 (1:1000), p-NF-κB p65 (1:1000); COX-2 (1:1000); TNFα (1:1000), IL-17 (1:1000), iNOS (1:1000) and β-actin (1:2000); all dilutions were in TBST supplemented with 1% BSA.
After washing with TBST, membranes were probed for 1 h with appropriate secondary antibody (HRP-conjugated goat anti-rabbit IgG antibody, 1:10 000 in TBST/1% BSA). Protein bands were then visualized using an enhanced chemiluminescence detection system (BioRad). The densities of the bands were measured using a BioRad Chem DOCTM XRS luminescent image analyzer and accompanying Imagelab [v.2.0.1] software.
Flow cytometric analyses of intracellular ROS levels
Levels of intracellular ROS production were directly measured using dichloro-dihydro-fluorescein diacetate (DCFH-DA) dye as described in Chen et al. (Citation2013). DCFH-DA is a non-fluorescent cell-permeable agent that enters cells and is deacetylated by non-specific esterase; the product is oxidized by ROS to yield fluorescent 2.7-dichlorofluorescein (DCF). Thus, fluorescence intensity is indicative of ROS levels in the cells. For the assay here, the RAW 264.7 cells that had been incubated with triphala or Indo for 24 h and then with LPS for a further 24 h were washed, harvested by scraping and then suspended in PBS containing 10 μM DCFH-DA for 30 min at 37 °C. The cells were then washed twice with ice-cold PBS and then analyzed by flow cytometry in a FACS Calibur system (Becton-Dickinson, San Diego, CA) at excitation-emission wavelengths of 488 and 525 nm, respectively. Fluorescent signal intensity was analyzed using CELLQuest software (v.3.0, Becton-Dickinson). A minimum of 10 000 events/sample was acquired for data analysis. Data were reported in terms of fluorescence intensity units (FIU).
In vivo studies
Studies of the effects of the triphala on adjuvant-induced arthritis in rodent hosts were previously performed by our laboratory (Rasool & Sabina Citation2007). However, details about specific molecular targets of effect of the extract remain, to date, unresolved. To both obtain information about this and to ascertain if many of the anti-inflammatory effects of triphala seen in the in vitro studies might translate to an in vivo scenario, the effects of the extract on select proteins of adjuvant-induced arthritic rats were evaluated here as well.
Animals
Wistar rats of either sex (125–150 g, 7-weeks-of-age) were procured from the Animal House of VIT University (Vellore, India). All rats were housed in a pathogen-free facility maintained at 25 ± 2 °C with a 44–45% relative humidity and a 12-h dark:light cycle. All rats had ad libitum access to a commercial balanced diet (Hindustan Lever Ltd, Mumbai, India) and filtered tap water. All animals were treated and cared for in accordance with guidelines recommended by the CPCSEA (Committee for the Purpose of Control and Supervision of Experiments on Animals), Government of India. All experimental protocols were approved by the Animal Ethics Committee of VIT University.
Experimental groups
Prior to the start of the experiments, rats were acclimated for 1 week. For the experiment, rats were randomly allocated into four groups, each comprised of six animals: Group I = Control rats administered saline, Group II = Adjuvant-induced arthritic rats, Group III = Arthritic rats to be administered triphala (100 mg/kg BW) and Group IV = Arthritic rats to be administered indomethacin (3 mg/kg BW). The doses of triphala used in this study were selected based on our preliminary studies (Kalaiselvan & Rasool Citation2015a,Citationb) with different dosages (50, 75 or 100 mg of triphala extract) that found that a dose of 100 mg/kg produced a significant anti-inflammatory effect (i.e. reduced paw swelling in adjuvant-induced arthritic animals). The dose of Indo was based upon a value previously reported in the literature (Rasool & Varalakshmi Citation2006).
Induction of arthritis and assessment
Arthritis was induced by a single intradermal injection of complete Freund’s adjuvant (CFA, 0.1 ml containing heat-killed Mycobacterium tuberculosis [10 mg/ml] in paraffin oil) into the footpad of the right hind paw. Triphala or indomethacin was administered intraperitoneally once daily for 8 days (from Day 11 to 18) after administration of the CFA. On Day 21, at the end of the experimental period, the animals were euthanized by cervical dislocation and their paw tissues recovered. Paw tissue lysates were prepared in ice-cold RIPA buffer mixed containing protease inhibitors and then analyzed for the protein expression of transcription factors (NF-κB p65 and p-NF-κB p65), inflammatory enzymes (COX-2 and iNOS), pro-inflammatory cytokines (TNFα and IL-17) and β-actin by Western blot analyses as described above.
Statistical analysis
All results were reported as mean ± SD. All statistical analysis was performed using InStat 3 software (GraphPad, San Diego, CA). Assessments of significant differences between groups were performed using a one-way analysis of variance (ANOVA), followed by a Student’s Newman-Keul’s test. A p-value < 0.05 implied significance.
Results
HPLC analyses of standard and triphala
The HPLC profile of triphala extract revealed peaks for five known major immunomodulatory constituents with retention times of 2.10, 2.95, 3.90, 5.13 and 7.08 min (). By comparison against standards, peaks associated with these retention times (and component percentage weight composition) in the samples were identified as, respectively, gallic acid (0.63% [w/w]), ascorbic acid (0.44% [w/w]), tannic acid (0.37% [w/w]), chebulagic acid (0.29% [w/w]) and chebulic acid (0.05% [w/w]) in each 1000 μg of sample. This indicated that these known immunomodulants comprised ∼ 2% of the extract utilized in these studies. The remainder of the materials were likely to have included, at much lower levels than the five above-noted compounds – ellagic acid, chebulinic acid, syringic acid, protocatechuic acid, catechins, epicatechin and epigallocatechin – based on previous analyses (Singh et al. Citation2008; Pawar et al. Citation2009; Jaijoy et al. Citation2010; Kumar et al. Citation2010; Nampoothiri et al. Citation2011).
Figure 1. HPLC chromatogram of triphala extract. Typical chromatogram of triphala exract prepared here. Key components were identified based on the identical retention times as those of the standards for (1) gallic, (2) ascorbic, (3) tannic, (4) chebulagic and (5) chebulic acids.
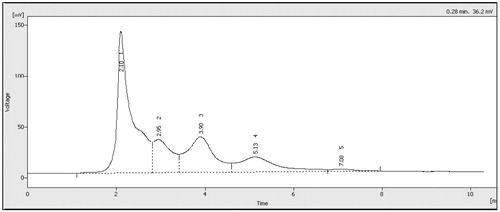
Re-analyses showed the composition of triphala extracts was not altered during each preparation of extracts for use in the experiments. The RSD (relative standard deviation) values calculated for the active constituents in the triphala extract at different times of preparation was < 5%; therefore, any minor variation in phytochemical concentrations did not likely have any significant impact on the biological activity observed therein. Further, any slight change in the retention time of a given eluted component in the extract could have been due to the presence of a conjugated form, i.e. a glycoside, that could easily be solubilized in situ/in cells.
In vitro studies
Effects of triphala extract on cell viability
Neither overt cytotoxicity nor changes in cell morphology were observed after a 24-h triphala extract treatment at up to a level of 500 μg/ml (data not shown). Based on these results, only triphala extract levels < 500 μg/ml were used hereafter in the studies.
Effects on lysosomal enzyme release
To ensure triphala extract inhibited release of lysosomal enzymes (acid phosphatase, β-galactosidase, N-Acetyl glucosaminidase, cathepsin D) by LPS-induced RAW 264.7 macrophages, the cells were treated with extract or indomethacin (Indo) prior to stimulation with LPS. The results indicated there was a significant increase in the activities of the assayed enzymes with LPS-induced cells compared to with unstimulated macrophages (). Triphala extract pre-treatment resulted in significant dose-related reductions in LPS-stimulated cells; levels at the highest dose approximated those seen with Indo-pre-treated cells and often were similar to those of the untreated specimens.
Table 2. Lysosomal enzyme release by LPS-treated RAW 264.7 macrophages.
Effects on NO, PGE2 and pro-inflammatory cytokine production
As shown in , LPS treatment caused increased production of NO and PGE2 compared to that by unstimulated macrophages. Pre-treatment with triphala extract (or Indo) caused a significant dose-related reduction in production of both products, with the use of the 300 μg triphala/ml dose bringing levels close to those seen with the untreated cells. Analysis of pro-inflammatory cytokine secretion by LPS-/unstimulated cells () revealed that, while there were low levels of basal TNFα, IL-1β, IL-6, MCP-1 and VEGF formation, stimulation with LPS induced significantly higher secretion of each protein. Pre-treatment with triphala extract significantly inhibited LPS-induced secretion of each protein; effects from the two highest triphala doses used often approximated that seen with Indo inhibitor.
Figure 2. Treatment effect on RAW 264.7 cell production of select inflammatory products. (A) PGE2; (B) NO. Values shown are means ± SD of data from three experiments. *Value significantly different (p < 0.05) vs all other treatment cells. Among all LPS-treated cells, values significantly different at p < 0.05: ‡vs LPS-only cells and #triphala-treated vs Indo-treated cells.
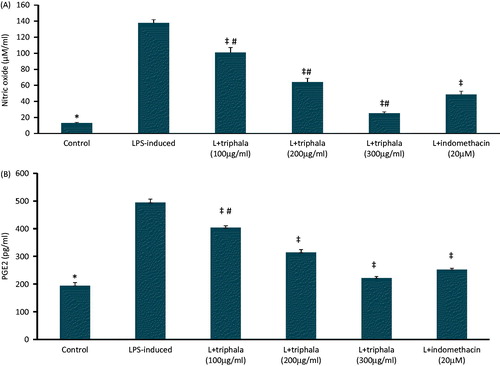
Figure 3. Treatment effect on production of select inflammatory proteins. (A) TNFα, (B) IL-1β, (C) IL-6, (D) MCP-1 and (E) VEGF. The values shown are means ± SD of data from three experiments. *Value significantly different (p < 0.05) vs all other treatment cells. Among all LPS-treated cells, values significantly different at p < 0.05: ‡vs LPS-only cells and #triphala-treated vs Indo-treated cells. Kit levels of sensitivity are provided in the Methods.
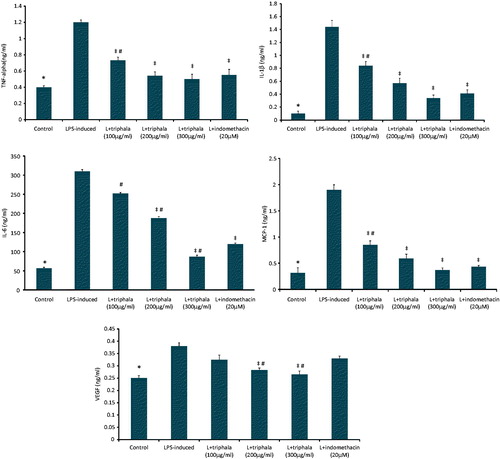
Effects on LPS-induced changes in expression of select genes
The effects of triphala on expression of the genes for TNFα, IL-1β, IL-6 and MCP-1, iNOS and COX-2 and transcription factor NF-κB-p65 LPS-stimulated RAW 264.7 cells were investigated by Real Time-PCR. As shown in the , the gene expression levels of each target gene was up-regulated in LPS-treated macrophages compared to in unstimulated cells. Triphala treatment, as well as Indo, markedly down-regulated these levels of induced gene expression in a dose-related manner.
Figure 4. Treatment effect on mRNA expression levels of select inflammatory proteins, NF-κB p65 and HO-1. (A) TNFα, (B) IL-1β, (C) IL-6, (D) MCP-1, (E) iNOS, (F) COX-2, (G) NFκB-p65 and (H) HO-1. Results are expressed as fold-change from the LPS only group. RQ values are calculated relative to GAPDH gene. Results shown are ± SD of data from three experiments. *Value significantly different (p < 0.05) vs all other treatment cells. Among all LPS-treated cells, values significantly different at p < 0.05: ‡vs LPS-only cells and #triphala-treated vs Indo-treated cells.
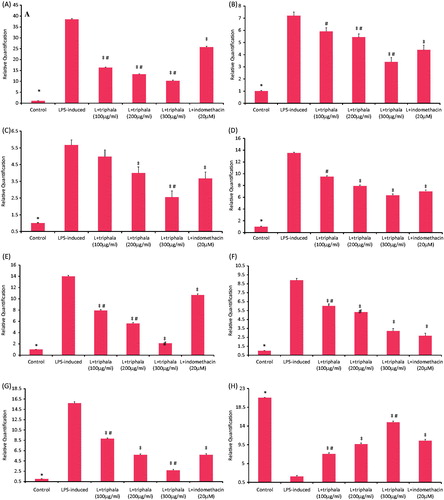
These LPS-treated cells were also examined to ascertain if triphala extract exerted anti-inflammatory actions through changes in HO-1 or NF-κB p65 transcription. As shown in , triphala treatment caused significant down-regulation in NF-κB p65 expression; conversely, triphala led to up-regulated HO-1 transcription (). With regard to these effects, again, the highest doses of triphala yielded outcomes similar to/greater than that from Indo.
Immunofluorescence assay
To begin to better understand mechanisms underlying effects of triphala extract on LPS-induced gene expression, changes in nuclear translocation of p-NF-κB p65 in the LPS-treated cells were evaluated. Immunofluore-scence analyses showed LPS stimulation caused increased translocation of p-NF-κB p65 from the cytoplasm into the nucleus () and that triphala extract pre-treatment significantly inhibited this outcome (as did Indo). To verify that the observed changes in gene expression outlined above were reflected at the post-translational level as well, the expression of COX-2 in these cells was also examined. The results indicated as with the gene data that LPS induced significant increases in COX-2 expression in the cells and that triphala extract (and Indo) reduced these increases ().
Figure 5. Treatment effect on p-NF-κB p65 nuclear translocation and COX-2 protein expression. Translocation was detected by immunofluorescence using (A) anti-p-NF-κB p65 (red) antibody or (B) anti-COX-2 (green) antibody; in each case, DAPI (blue) staining was also performed. Representative images from each treatment set are shown. Magnification = 40×.
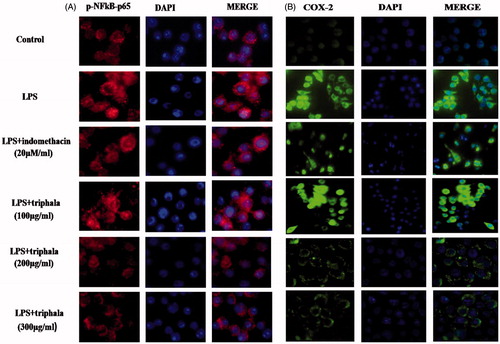
Effects on LPS-induced NF-κB signaling
To discern if the noted suppressive effects of triphala extract on LPS-stimulated pro-inflammatory cytokines/enzymes were possibly due to an impact on activation of NF-κB signaling, BAY 11-7082 (an IκB kinase inhibitor) was employed. As shown in , Western blot analyses clearly showed LPS-stimulated cells had an elevated expression of NF-κB p65 and p-NF-κB p65 proteins compared to that seen with control cells. Extract pre-treatment led to decreased expression of NF-κB p65 and p-NF-κB p65 in the cells, with the 300 μg/ml dose causing an expression akin to that attained with Indo. Use of BAY 11-7082 near-completely blocked p-NF-κB p65 protein expression in the cells. These patterns of change in NF-κB p65 and p-NF-κB p65 expression in the cells was mirrored by changes in expression of TNF, iNOS and IL-17 by the cells (). Interestingly, the effects from triphala extract, Indo and BAY 11-7082 did not translate as well, with use of the IκB kinase inhibitor having no apparent significant effect on the COX-2 formation induced by LPS.
Figure 6. Treatment effect on expression of select proteins. (A) Relative expression values for NF-κB p65 and p-NF-κB p65 and for (B) IL-17, TNFα, iNOS and COX-2, all based on band intensities in Western blots. Values shown are means ± SD of data from three experiments. *Value significantly different (p < 0.05) vs all other treatment cells. Among all LPS-treated cells, values significantly different at p < 0.05: ‡vs LPS-only cells, #triphala-treated vs Indo-treated cells and @triphala-treated vs Bay-treated cells.
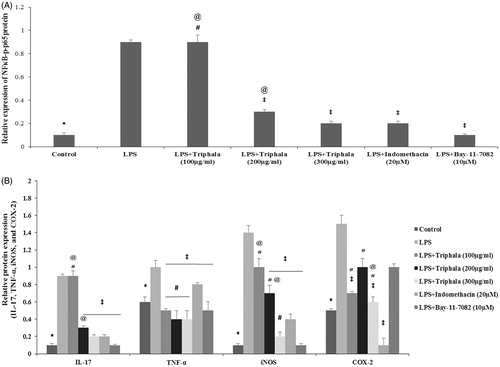
Effects on LPS-induced intracellular free radical production
Over-production of free radicals during inflammatory processes are involved in signal transduction and NF-κB activation. The analyses of the effects of triphala on intracellular free radical production in LPS-stimulated RAW 264.7 cells indicated that production was increased compared to that with control cells (). DCFH-DA fluorescence intensity in LPS-treated cells was ≈12 fluorescence intensity units (FIU) (≈48%; p ≤ 0.05) greater than that in control cells. Extract pre-treatment significantly diminished the LPS-induced radical production, with the DCFH-DA fluorescence decreasing by 9.1, 12.0 and ≈19.0 FIU relative to that in LPS-only treated cells (≈24, 32 and 51%; all p ≤ 0.05). Indo pre-treatment only had a nominal mitigating effect on this parameter (DCFH-DA fluorescence decreased by 3.8 points [≈10%; p > 0.05]).
Figure 7. Treatment effect on intracellular free radical formation. (A) Fluorescence intensity units (FIU) shown are means ± SD of data from six experiments. *Value significantly different vs LPS-only treated cell and vs LPS + Indo-treated cells (p < 0.05). Among all LPS-treated cells, values significantly different at p < 0.05: ‡vs LPS-only cells and #triphala-treated vs Indo-treated cells.
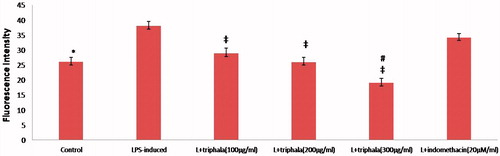
In vivo studies
Western blot analysis
To ascertain if many of the anti-inflammatory effects of triphala extract seen in the in vitro studies might translate to the in vivo scenario, the effects of the agent on select proteins in adjuvant-induced arthritic rats were evaluated. Specifically, expression levels of NF-κB p65, p-NF-κB p65, IL-17, COX-2 and RANKL were evaluated in paw tissues of adjuvant-induced arthritic rats by Western blot analysis. As shown in , the expression of NF-κB p65, p-NF-κB p65, IL-17, COX-2 and RANKL proteins were significantly elevated in the paw tissues of the arthritic rats when compared with in tissues from control rats. Triphala extract treatment (administered IP once daily for 8 days [from Day 11 to 18] after administration of the CFA [at 100 mg/kg]) significantly decreased expression levels of p-NFκB p65, IL-17, COX-2 and RANKL proteins in the arthritic rat tissues; except for with IL-17, effects were often on par with that induced by use of Indo.
Figure 8. Treatment effect on arthritic rat paw levels of select proteins. (A) Relative expression values for NF-κB p65 and p-NF-κB p65 and for (B) IL-17, RANKL and COX-2, all based on band intensities in Western blots. Values shown are means ± SD of data from three experiments. *Value significantly different (p < 0.05) vs all other treatments. Among all AIA rats’ samples, values significantly different at p < 0.05: ‡vs AIA-only hosts and #triphala-treated vs Indo-treated hosts.
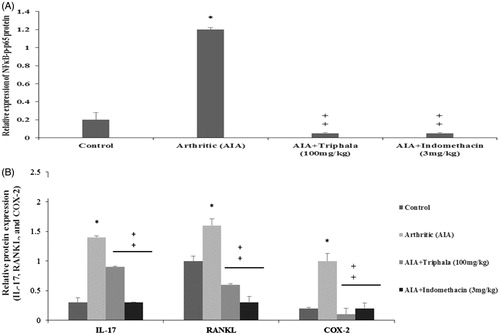
Discussion
Our earlier reports showed that triphala extract had promising in vivo anti-inflammatory and anti-arthritic effects in adjuvant-induced arthritic rats (Kalaiselvan & Rasool Citation2015a). To explore anti-inflammatory molecular mechanisms, the present study focused on the inhibitory effects of the extract on pro-inflammatory cytokine, inflammatory enzyme, and inflammatory factor production by LPS-induced RAW 264.7, as well as their expression at the mRNA level. In addition, to investigate if the inhibitory action of triphala extract on inflammatory factor/cytokine production was mediated by inhibition of NF-κB pathways and/or via HO-1 up-regulation, NF-κB activation and HO-1 expression in LPS-induced cells was also analyzed.
HPLC profiling of the triphala extract performed here revealed the presence of poly-phenols including ascorbic, tannic, chebulagic, chebulic and gallic acids, with the latter being the predominant component. Each of these active principles have been reported to impart various biological effects, including immunomodulatory and both anti-inflammatory and anti-oxidant effects (Ahn et al. Citation2015; Liu et al. Citation2015). In addition to these components, previous analyses indicated there was a presence of ellagic acid, chebulinic acid, syringic acid, protocatechuic acid, catechins, epicatechin and epigallocatechin, but at minimal concentrations (Nampoothiri et al. Citation2011; Pawar et al. Citation2009). Ahn et al. (Citation2004), Byun et al. (Citation2011,Citation2012) and Rosillo et al. (Citation2012) reported immunomodulatory effects from ellagic acid and epigallocatechin in murine dendritic cells and in a murine model. However, in the triphala extract, these agents did not likely render significant activity due to their presence at very low levels. Nevertheless, the possibility remains that, in concert with the five major agents noted above, there could have been some contributions. Further studies with the individual agents (each alone and in combination with ascorbic, tannic, chebulagic, chebulic and/or gallic acids) are needed to clarify potential additive or synergistic (or even potentially inhibitory) effects from such combinations.
With respect to the predominant component here, it is known that gallic acid can help to reduce inflammation, in part, by causing reductions in DNA binding with NF-κB in cells treated with LPS, thereby leading to a subsequent lessening of expression of pro-inflammatory mediators. Further, gallic acid can inhibit TNFα and IL-6 formation/release by human monocytes secondary to an induced suppression of p65 acetylation (Choi et al. Citation2009). In addition, other earlier studies had already noted that some of the detected components (and other less-present individual components like 4-O-methylgallic acids and bellericanin) were able to affect transcription of genes for TNFα, IL-1β, IL-6, COX-2 and even NF-κB (Na et al. Citation2006; Yu et al. Citation2007; Sabina & Rasool Citation2008; Zhao et al. Citation2008). Thus, agents within the triphala extract had multiple means by which to impact on genes/pathways needed by activated macrophages to contribute to inflammation.
Normally, exposure to LPS induces macrophage production of several inflammatory cytokines (e.g. TNFα, IL-1β, IL-6, MCP-1, VEGF) and mediators like NO and PGE2 (produced by inducible nitric oxide synthase [iNOS] and COX-2, respectively). Several reports have noted that NO at high levels causes cell death (Ahmad et al. Citation2009) and contributes to inflammatory/autoimmune diseases (Lee et al. Citation2005b). Similarly, PGE2, like many prostaglandins, also plays a major role in inflammation (Salvemini et al. Citation2013). Therefore, inhibition of excessive PGE2 and NO generation by blocking COX-2 and/or iNOS could be a meaningful strategy to treat inflammation-based diseases. The present findings suggested to us triphala mediated reductions in NO and PGE2 at both the transcriptional and translational level.
There are many factors that control PGE2 and NO expression. In this regard, NF-κB plays a central role in regulating genes encoding mediators, including iNOS and COX-2 during inflammation. Following NF-κB p65 translocation into the nucleus, expression of iNOS and COX-2 and other pro-inflammatory cytokine genes (like for IL-6 and TNFα) is affected in macrophages (Lee et al. Citation2009). Accordingly, modulation of NF-κB activity also presents a promising target for treatment of inflammation-related disorders. Here, it was seen that p-NF-κB p65 translocation and its mRNA expression in LPS-treated RAW 264.7 macrophages was strongly inhibited by triphala treatment. This outcome (as well as reduced COX-2 expression) was in accord with earlier reports that chebulagic acid (triphala component) caused COX-2 suppression at both the transcription and translational level by inhibition of NF-κB-mediated pathways (Reddy & Reddanna Citation2009; Karlsson et al. Citation2010).
The present study also showed triphala extract significantly inhibited the mRNA expression/cell production of TNFα, IL-1β, IL-6, MCP-1 and VEGF by activated macrophages. Since NF-κB-mediated signaling strongly regulates the release of cytokines/inflammatory mediators in activated macrophages (Hwang et al. Citation2010), to confirm that effects of triphala were being mediated through the NF-κB pathway, studies using BAY 11-7082 (inhibitor of IκB kinase) on LPS-stimulated production of iNOS, COX-2, IL-17 and TNFα were performed in parallel here. The Western blot analyses showed that LPS-stimulated RAW 264.7 cells had elevated NF-κB p65 and p-NF-κBp65 expression and, as expected, enhanced expression of TNFα, IL-17, iNOS and COX-2 proteins. In contrast, triphala treatment decreased the induced expressions of NF-κB p65 and p-NF-κB p65 in the cells (and subsequently suppressed expression of TNFα, IL-17, iNOS and COX-2). These outcomes were in line with those seen to be induced by the BAY 11-7082. These outcomes were in accordance with earlier reports that gallic, chebulagic and tannic acid (major triphala components here) caused a modulating effects on NF-κB p65 and p-NF-κBp65 expression (Reddy & Reddanna Citation2009; Ho et al. Citation2010; Karuppagounder et al. Citation2015). In addition, even some of the minor components in the extract, e.g. ellagic acid and epigallocatechin, are known to inhibit expression of TLR4, TNFα, IL-6 and IL-1β by blocking NF-κB-mediated inflammatory pathways (Ahn et al. Citation2004; Byun et al. Citation2011, Citation2012; Rosillo et al. Citation2012). Taken in total, these results indicated strongly that the suppressive effect of triphala on inflammatory mediator production/expression could be via inhibition of NF-κB signaling.
Macrophages stimulated by LPS undergo membrane damage due to increasing oxidative stress; this leads to an eventual rupture of lysosomal membranes/discharge of lysosomal enzymes (array of hydrolytic enzymes that hydrolyze proteins, nucleic acids, polysaccharides, lipids and phospholipids (Pragasam & Rasool Citation2013)) and reactive oxygen species (ROS)/free radicals, each causing amplification of the inflammatory responses. As this release of lysosomal enzymes/ROS plays a critical role in tissue injury, a reduction in their release would also be beneficial in mitigating inflammatory responses. Here, LPS-stimulated macrophages showed excessive release of lysosomal enzymes and free radicals compared to control cells. Interestingly, triphala treatment caused significant reduction in these releases. This indicated triphala seemed to impart a membrane stabilizing/radical scavenging effect similar to one already noted in Cheng et al. (Citation2003), Hari Kumar et al. (Citation2004) and Lee et al. (Citation2005a). Moreover, the present results indicating triphala exhibited anti-radical effects (by limiting the intracellular ROS generation) were in concordance with studies of protective effects of gallic acid against oxidative injury (Chen et al. Citation2013).
Heme oxygenase-1 (HO-1), a rate-limiting enzyme in catabolism of heme to biliverdin, free iron and carbon monoxide (CO), has been implicated in protection against oxidative stress (Willis et al. Citation1996). It was reported that over-expression of HO-1 counteracts cytotoxic, pro-oxidative/-inflammatory effects caused by heme accumulation by causing a down-regulation in levels of inflammatory adhesion molecules, enzymes and cytokines and causing an abrogation of leukocytes to inflamed tissues (Wagener et al. 2003). The present triphala study results were in keeping with those of other studies showing several phytochemicals might be useful therapeutics against inflammatory disorders as they cause up-regulated HO-1 expression.
Our earlier studies already confirmed anti-inflammatory effects of triphala extract in arthritic hosts. It is established that, during arthritis, activated immune cells produce TNFα, IL-6, IL-17 and RANKL to help mediate synovial inflammation and production of inflammatory enzymes like COX-2 and iNOS (as well as matrix metalloproteinases) via increased activation of NF-κB (Sulthana & Rasool Citation2015). Specifically, those earlier studies documented that use of the extract led to changes in IL-17, RANKL and COX-2 mRNA expression (evaluated by real time-PCR) in paw tissues of adjuvant-induced arthritic rats (Kalaiselvan & Rasool Citation2015a,Citationb). To investigate if the in vitro effects from triphala extract on cell expression of NF-κB p65 and p-NF-κB p65—as well as of key participants involved in arthritic/inflammatory responses in situ (IL-17, COX-2 and RANKL, whose expression each either impact on/are impacted by expression/activation of NF-κB) also took place in arthritic hosts, Western blot analyses of NF-κB p65, p-NF-κB p65, IL-17, COX-2 and RANKL levels in the paw tissues were performed here.
RANKL was chosen for examination in that osteoclastic activity is triggered via activation of osteoclast surface-bound receptor activator of NF-κB (RANK) (Geusens Citation2012). Over-production of RANKL is implicated in a variety of degenerative bone diseases, including arthritis. Thus, if the extract reduced RANKL expression, it would be expected there would be less NF-κB activation at the site of inflammation (i.e. paws). IL-17 was chosen for examination in that it induces production of many other cytokines (IL-6, G-CSF, GM-CSF, IL-1β, TGFβ, TNFα), chemokines (IL-8, GROα, MCP-1) and prostaglandins from many cell types (fibroblasts, macrophages, etc.). As a result, IL-17 is key in many immune/autoimmune diseases, including arthritis. It is also known that NF-κB (along with STAT3) signal pathways are required for inducible IL-17 production (Cho et al. Citation2006). Thus, if the extract reduced NF-κB activation/expression, less local IL-17 at the site of inflammation (i.e. paws) would be expected. Similarly, with COX-2, p38/NF-κB activation/recruitment are required in inflammatory responses for increased COX-2 formation (Ulivi et al. Citation2008). Accordingly, as with IL-17, any extract-induced reductions in NF-κB activation/expression would then be expected to result in less local COX-2 in the arthritic rats’ paws.
The results showed the extract meaningfully reduced the expression of each of the NF-κB-expression/activation-related proteins in the paw tissues of the arthritic rats. Similarly, the expression levels of NF-κB p65 and p-NF-κB p65 were also strongly reduced by treatment of the hosts with the triphala extract. While use of Western blots (which are semi-quantitative at best) were helpful in showing that in situ outcomes not only confirmed the translatability of the in vitro study data, appropriate measures of IL-17 and RANKL expression (by ELISA) and of COX-2 activity (enzymatically) are underway to bolster these findings. Notwithstanding those results, these outcomes in the adjuvant-induced arthritic rats provide sufficient support for our hypothesis that triphala extract inhibited the generation of inflammatory mediators in situ, most likely through inhibition of NF-κB signaling pathways.
Conclusions
The data here demonstrated that a triphala extract efficiently inhibited production/gene expression of inflammatory enzymes, pro-inflammatory cytokines and inflammatory mediators in LPS-stimulated RAW 264.7 macrophage cells in a manner similar to that of the drug indomethacin. The results showed these effects were, in part, via up-regulation of HO-1 and suppression of transcription factor NF-κB nuclear translocation. The animal model studies also confirmed that triphala exhibited anti-inflammatory effects by reducing expression of inflammatory mediators via inhibition of NF-κB activation. Taken together, these findings demonstrate that triphala extract has potential anti-inflammatory parameters that could make it useful for the treatment of several inflammatory disorders, including rheumatoid arthritis.
Acknowledgments
The authors are grateful for the financial assistance (59/2/2011/BMS/TRM-AD-HOC Project) provided by the Indian Council of Medical Research, Government of India, to carry out this research.
Disclosure statement
The authors report no conflicts of interest. The authors alone are responsible for the content and writing of the paper.
References
- Ahmad R, Rasheed Z, Ahsan H. 2009. Biochemical and cellular toxicology of peroxy-nitrate implications in cell death and autoimmune phenomenon. Immunopharmacol Immunotoxicol. 31:388–396.
- Ahn CB, Jung WK, Park SJ, Kim YT, Kim WS, Je JY. 2015. Gallic acid-γ-chitosan modulates inflammatory responses in LPS-stimulated RAW 264.7 cells via NF-κB, AP-1, and MAPK pathways Inflammation. [Epub ahead of print]. doi: 10.1007/s10753-015-0258-2.
- Ahn SC, Kim GY, Kim JH, Baik SW, Han MK, Lee HJ, Moon DO, Lee CM, Kang JH, Kim BH. 2004. Epigallocatechin-3-gallate, constituent of green tea, suppresses the LPS-induced phenotypic and functional maturation of murine dendritic cells through inhibition of mitogen-activated protein kinases and NF-κB. Biochem Biophys Res Commun. 313:148–55.
- Biber J, Stieger B, Haase W, Murer H. 1981. A high yield, preparation of rat kidney brush-border membranes, different behaviors of lysosomal markers. Biochim Biophys Acta. 647:169–176.
- Biswas SK, Lewis CE. 2010. NF-κB as a central regulator of macrophage function in tumors. J Leukocyte Biol. 88:877–884.
- Byun EB, Choi HG, Sung NY, Byun EH. 2012. Green tea polyphenol epigallocatechin-3-gallate inhibits TLR4 signaling through the 67-kDa laminin receptor on LPS-stimulated dendritic cells. Biochem Biophys Res Commun. 426:480–485.
- Byun EH, Omura T, Yamada K, Tachibana H. 2011. Green tea polyphenol epigallocate-chin-3-gallate inhibits TLR2 signaling induced by peptidoglycan through the polyphenol sensing molecule 67-kDa laminin receptor. FEBS Lett. 9:814–820.
- Chen CY, Chen KC, Yang TY, Liu HC, Hsu SL. 2013. Gallic acid induces a reactive oxygen species-provoked c-jun NH2-terminal kinase-dependent apoptosis in lung fibroblasts. Evid-Based Complement Alt Med. 2013:1–12.
- Cheng HY, Lin TC, Yu KH, Yang CM, Lin CC. 2003. Anti-oxidant and free radical scavenging activities of Terminalia chebula. Biol Pharm Bull. 26:1331–1335.
- Cho ML, Kang JW, Moon YM, Nam HJ, Jhun JY, Heo SB, Jin HT, Min SY, Ju JH, Park KS, et al. 2006. STAT3 and NF- κB signal pathway is required for IL-23-mediated IL-17 production in spontaneous arthritis animal model IL-1 receptor antagonist-deficient mice. J Immunol. 176:5652–5661.
- Choi KC, Lee YH, Jung MG, Kwon SH, Kim MJ, Jun WJ, Lee J, Lee JM, Yoon HG. 2009. Gallic acid suppresses lipopolysaccharide-induced NF- κB signaling by preventing RelA acetylation in A549 lung cancer cells. Mol Cancer Res. 7:2011–2021.
- Choudhari AS, Raina P, Deshpande MM, Wali AG, Zanwar A, Bodhankar SL, Kaul-Ghanekar R. 2013. Evaluating the anti-inflammatory potential of Tectaria cicutaria L. rhizome extract in vitro as well as in vivo. J Ethnopharmacol. 150:215–222.
- El-Mekkawy S, Meselhy MR, Kusumoto I, Kadota TS, Hattori M, Namba T. 1995. Inhibitory effects of Egyptian folk medicines on human immunodeficiency virus (HIV) reverse transcriptase. Chem Pharm Bull. 43:641–648.
- Geusens P. 2012. The role of RANK ligand/osteoprotegerin in rheumatoid arthritis. Ther Adv Musculoskel Dis. 4:225–233.
- Grivennikov SI, Greten FR, Karin M. 2010. Immunity, inflammation, and cancer. Cell. 140:883–899.
- Hari Kumar KB, Sabu MC, Lima PS, Kuttan R. 2004. Modulation of hematopoetic system and anti-oxidant enzymes by Emblica officinalis Gaertn and its protective role against γ-radiation-induced damage in mice. J Radiation Res (Tokyo). 45:549–555.
- Ho HH, Chang CS, Ho WC, Liao SY, Wu CH, Wang CJ. 2010. Anti-metastasis effects of gallic acid on gastric cancer cells involve inhibition of NF-κB activity and down-regulation of PI3K/AKT/small GTPase signals. Food Chem Toxicol. 48:2508–2516.
- Hwang JM, Yu JY, Jang YO, Kim BT, Hwang KJ, Jeon YM, Lee JC. 2010. A phenolic acid phenethyl urea compound inhibits lipopolysaccharide-induced production of nitric oxide and pro-inflammatory cytokines in cell culture. Intl Immunopharmacol. 10:526–532.
- Jagetia GC, Baliga MS, Malagi KJ, Kamath SM. 2002. Evaluation of the radioprotective effect of triphala in mice exposed to γ-radiation. Phytomedicine. 9:99–108.
- Jaijoy K, Soonthornchareonnon N, Panthong A, Sireeratawong S. 2010. Anti-inflammatory and analgesic activities of the water extract from the fruit of Phyllanthus emblica Linn. Int J Appl Res Nat Prod. 3:28–35.
- Kalaiselvan S, Rasool M. 2015b. Triphala exhibits anti-arthritic effect by ameliorating bone and cartilage degradation in adjuvant-induced arthritis rats. Immunol Invest. 44:411–426.
- Kalaiselvan S, Rasool MK. 2015a. The anti-inflammatory effect of triphala in adjuvant-induced arthritis in rats. Pharm Biol. 53:51–60.
- Karlsson S, Nanberg E, Fjaeraa C, Wijkander J. 2010. Ellagic acid inhibits LPS-induced expression of enzymes involved in the synthesis of PGE2 in human monocytes. Br J Nutr. 103:1109–1119.
- Karuppagounder V, Arumugam S, Thandavarayan RA, Pitchaimani V, Sreedhar R, Afrin R, Harima M, Suzuki H, Nomoto M, Miyashita S, et al. 2015. Tannic acid modulates NF-κB signaling pathway and skin inflammation in NC/Nga mice through PPARγ expression. Cytokine. 76:206–213.
- Kaur S, Arora S, Kaur K, Kumar S. 2002. The in vitro anti-mutagenic activity of triphala – an Indian herbal drug. Food Chem Toxicol. 40:527–534.
- Kaur S, Michael H, Arora S. 2005. The in vitro cytotoxic and apoptotic activity of triphala – an Indian herbal drug. J Ethnopharmacol. 97:15–20.
- King J. 1965. The hydrolases-acid and alkaline phosphatases In: Van D, editor. Practical clinical enzymology. London: Van Nostrand Company Ltd, p. 191–208.
- Kumar MS, Kirubanandan S, Sripriya R, Sehgal PK. 2010. Triphala-incorporated collagen sponge – a smart biomaterial for infected dermal wound healing. J Surg Res. 158:162–170.
- Lee MY, Park BY, Kwon OK, Yuk JE, Oh SR, Kim HS, Lee HK, Ahn KS. 2009. Anti-inflammatory activity of (-) aptosimon isolated from Daphne genkwa in RAW 264.7 cells. Intl Immunopharmacol. 9:878–885.
- Lee SI, Hyun PM, Kim SH, Kim KS, Lee SK, Kim BS, Maeng PJ, Lim JS. 2005a. Suppression of the onset and progression of collagen induced arthritis by chebulagic acid screened from a natural product library. Arthritis Rheum. 52:345–353.
- Lee YS, Han OK, Park CW, Yang CH, Jeon TW, Yoo WK, Kim SH, Kim HJ. 2005b. Pro-inflammatory cytokine gene expression and nitric oxide regulation of aqueous extracted Astragali radix in RAW 264.7 macrophage cells. J Ethnopharmacol. 100:289–294.
- Li F, Nitteranon V, Tang X, Liang J, Zhang G, Parkin KL, Hu Q. 2012. In vitro anti-oxidant and anti-inflammatory activities of 1-dehydro-[6]-gingerdione, 6-shogaol, 6-dehydroshogaol and hexahydrocurcumin. Food Chem. 135:332–337.
- Liu Y, Bao L, Xuan L, Song B, Lin L, Han H. 2015. Chebulagic acid inhibits the LPS-induced expression of NF-κB and IL-1β in endothelial cells by suppressing MAPK activation. Exp Ther Med. 10:263–268.
- Lowry OH, Rosebrough NJ, Farr AL, Randall RJ. 1951. Protein measurement with the Folin phenol reagent. J Biol Chem. 193:265–275.
- Maruhn D. 1976. Rapid colorimetric assay of β-galactosidase and N-acetyl-β-glucosaminidase in human urine. Clin Chim Acta 73:453–461.
- Na HJ, Lee G, Oh HY, Jeon KS, Kwon HJ, Ha KS, Lee H, Kwon YG, Kim YM. 2006. 4-O-Methylgallic acid suppresses inflammation-associated gene expression by inhibition of redox-based NF-κB activation. Intl Immunopharmacol. 6:1597–1608.
- Nampoothiri SV, Prathapan A, Cherian OL, Raghu KG, Venugopalan VV, Sundaresan A. 2011. In vitro anti-oxidant and inhibitory potential of Terminalia bellerica and Emblica officinalis fruits against LDL oxidation and key enzymes linked to Type 2 diabetes. Food Chem Toxicol. 49:125–131.
- Pawar V, Lahorkar P, Anantha Narayana DB. 2009. Development of RP-HPLC method for analysis of triphala curna and its applicability to test variations in triphala curna preparations. Indian J Pharm Sci. 71:382–386.
- Pragasam SJ, Rasool M. 2013. Dietary component p-coumaric acid suppresses mono sodium urate crystal-induced inflammation in rats. Inflamm Res. 62:489–498.
- Rasool M, Varalakshmi P. 2006. Suppressive effect of Withania somnifera root powder on experimental gouty arthritis: an in vivo and in vitro study. Chem Biol Interact. 164:174–180.
- Rasool M, Sabina EP. 2007. Anti-inflammatory effect of the Indian Ayurvedic herbal formulation triphala on adjuvant-induced arthritis in mice. Phytother Res. 21:889–894.
- Reddy DB, Reddanna P. 2009. Chebulagic acid (CA) attenuates LPS-induced inflammation by suppressing NF-κB and MAPK activation in RAW 264.7 macrophages. Biochem Biophys Res Commun. 381:112–117.
- Rosenblit PD, Metzyer RP, Wick AN. 1974. Effect of streptozotocin diabetes on acid phosphatase and selected glycosidase activities of serum and various rat organs. Proc Soc Exp Biol Med. 14:244–248.
- Rosillo MA, Sánchez-Hidalgo M, Cárdeno A, Aparicio-Soto M, Sánchez-Fidalgo S, Villegas I, de la Lastra CA. 2012. Dietary supple-mentation of ellagic acid-enriched pomegranate extract attenuates chronic colonic inflamma-tion in rats. Pharmacol Res. 66:235–242.
- Ryter SW, Otterbein LE, Morse D, Choi AM. 2002. Heme oxygenase/carbon monoxide signaling pathways: regulation and functional significance. Mol Cell Biochem. 234:249–263.
- Sabina EP, Rasool M. 2008. An in vivo and in vitro potential of Indian ayurvedic herbal formulation triphala on experimental gout arthritis in mice. Vasc Pharmacol. 48:14–20.
- Sabina EP, Rasool MK, Mathew L. 2009. In vivo and in vitro immunomodulatory effects of Indian Ayurvedic herbal formulation triphala on experimentally-induced inflammation. Pharmacologyonline. 2:840–849.
- Sabu MC, Kuttan R. 2002. Anti-diabetic activity of medicinal plants and its relationship with their antioxidant property. J Ethnopharmacol. 81:155–160.
- Sandhyaa T, Lathikaa KM, Pandeyb BN, Mishraa KP. 2006. Potential of traditional Ayurvedic formulation, triphala, as a novel anti-cancer drug. Cancer Lett. 231:206–214.
- Salvemini D, Kim SF, Mollace V. 2013. Reciprocal regulation of the nitric oxide and cyclo-oxygenase pathway in pathophysiology: relevance and clinical implications. Am J Physiol. 304:R473–R487.
- Singh DP, Govindarajan R, Rawat AK. 2008. High-performance liquid chromatography as a tool for the chemical standardisation of triphala – an Ayurvedic formulation. Phytochem Anal. 19:164–168.
- Sulthana F, Rasool M. 2015. A novel therapeutic approach targeting rheumatoid arthritis by combined administration of morin, a dietary flavanol and non-steroidal anti-inflammatory drug indomethacin with reference to pro-inflammatory cytokines, inflammatory enzymes, RANKL and transcription factors. Chem-Biol Interactions. 230:58–70.
- Takagi N, Sanashiro T. 1996. Health foods containing anti-oxidative and anti-allergy food materials. Japan Kokai Tokkyo Hoho. 70:1000.
- Ulivi V, Giannoni P, Gentili C, Cancedda R, Descalzi F. 2008. p38/NF-κB-dependent expression of COX-2 during differentiation and inflammatory response of chondrocytes. J Cell Biochem. 104:1393–1406.
- Wagener FA, Volk HD, Willis D, Abraham NG, Soares MP, Adema GJ, Figdor CG. 2003. Different faces of the heme-heme oxygenase system in inflammation. Pharmacol Rev. 55:551–571.
- Wang QS, Cui YL, Wang YF, Chi W. 2011. Effects of compounds from Bi-Qi capsule on the expression of inflammatory mediators in lipopolysaccharide-stimulated RAW 264.7 macrophages. J Ethnopharmacol. 136:480–487.
- Willis D, Moore AR, Frederick R, Willoughby DA. 1996. Heme oxygenase: a novel target for the modulation of inflammatory response. Nat Med. 2:87–93.
- Yu YM, Wang ZH, Liu CH, Chen CS. 2007. Ellagic acid inhibits IL-1β-induced cell adhesion molecule expression in human umbilical vein endothelial cells. Br J Nutr. 97:692–698.
- Zhao L, Zhang SL, Tao JY, Pang R, Jin F, Guo YJ, Dong JH, Ye P, Zhao HY, Zheng GH. 2008. Preliminary exploration on anti-inflammatory mechanism of corilagin (β1-ogalloyl- 3, 6-(R)-hexahydroxydiphenoyl-D-glucose) in vitro. Intl Immunopharmacol. 8:1059–1064.