Abstract
A new peroxidase biosensor was developed using cysteamine–palladium complex-modified gold electrode. The principle of the measurements is based on monitoring increase in the oxidation potential of palladium complex (at + 0.47 V vs Ag/AgCl) using amperometric detection. In the optimization studies of the biosensor, effects of enzyme amount, palladium complex amount, and duration of SAM formation on biosensor responses were investigated to optimize the bioactive layer. The biosensor has a fast response time of less than 10 s to hydrogen peroxide (H2O2), with a linear range of 5.0 × 10− 6 to 150 × 10− 6 M and a detection limit of 3.38 × 10− 6 M.
Introduction
The accurate and sensitive determination of hydrogen peroxide (H2O2) is very important in many fields including industry, environmental protection, and clinical control (Bartlett et al. Citation1998, Wang et al. Citation1993, Sellers Citation1980). There are many techniques such as titrimetry (Brestovisky et al. Citation1983), spectrophotometry (Matsubara et al. Citation1992), chemiluminescence (Hanaoka et al. Citation2001, Nakashima et al. Citation1991), and electrochemistry (Li et al. Citation1996, Garguilo et al. Citation1993, Xiao et al. Citation1999) for the detection of H2O2. Among these techniques, electrochemical methods, especially amperometric biosensors based on peroxidase enzymes, are very important for the determination of H2O2 because of its high sensitivity (Zhang and Oyama Citation2005, Ferapontova et al. Citation2002, Wang et al. Citation2009, Hong and Dai Citation2009). In the fabrication of biosensors, the immobilization of enzymes is the most important step. Several immobilization methods have been considered such as entrapment in a carbon-paste electrode (Grigor et al. Citation2010), adsorption onto activated carbon electrodes (Gorton et al. Citation1999), adsorption on thiol-modified gold electrodes (Gaspar et al. Citation2001), entrapment in a sol gel or hydrogel (Li et al. Citation1996, Wang et al. Citation2000), and entrapment in an electropolymerized film on the electrode surface (Razola et al. Citation2002, Cosnier Citation1999, Mala Ekanayake et al. Citation2008, Şenel et al. Citation2010). In the biosensor construction ease of fabrication, efficient retention of enzyme activity, and stability have to be considered. To improve the performance of the biosensor, it is preferable to find an easy-handling immobilization method. Self-assembled monolayers (SAMs) are highly ordered molecular assemblies formed by chemisorption of functionalized molecules on the metal or metal oxide surface, and organize themselves laterally. The most widely studied SAM systems have been formed by chemisorption of alkanethiols on gold, silver, or copper metal surfaces (Yan et al. Citation2004). Electrical communication between redox sites of enzyme and working electrode is the basis for developing various amperometric biosensors (Heller Citation1990, Vreeke et al. Citation1992). Nevertheless, because their redox centers are generally located deep inside the proteins, direct electron transfer is usually difficult with most enzymes. Both the sensitivity and the application of these unmediated biosensors have been limited due to the slow electron transfer rate. Therefore, suitable redox mediators are preferable to establish an electrical connection between redox centers of the enzymes and the working electrode.
In this study, a new electroactive palladium complex ([Pd(μ-OAc)(ppy)]2, ppy: 2-phenylpyridine, PhMe) has been used as a donor molecule in the enzymatic reaction of peroxidase enzyme.
Materials and methods
Chemicals and apparatus
Palladium complex was synthesized in our laboratory as described below. Horseradish peroxidase, cysteamine, glutaraldehyde (25%), H2O2, and all other chemicals were purchased from Sigma Chemical Co. (USA). 1-Methylimidazole was purchased from Merck. All solutions used in the experiments were prepared just before their use.
In the experiments PalmSens potentiostat (the Netherlands), a three-electrode system from CH Instruments (USA) that contains a CHI 102 model gold-working electrode, a CHI 111 model Ag/AgCl reference electrode, and a CHI 115 model platinum wire counter electrode, Gilson P100 and P1000 automatic pipettes (France), IKA magnetic stirrer (Germany), and Nuve model thermostat (TR) were used.
Synthesis of novel palladium complex
Quaternization of methylimidazole (1) by methyl-substituted benzyl bromide afforded imidazolium salt (2) which was converted to (κ2-C,N)-palladacycles bearing imidazol-2-ylidenes (3), by in situ deprotonation, using the bridged palladacycle, [Pd(μ-OAc)(ppy)]2, ppy: 2-phenylpyridine (Günay et al. Citation2009). The general route to the N-heterocyclic ligand (NHC) precursors and palladacyclic complexes is shown in . Palladium dimer and benzyl bromide derivative were prepared according to the literature (Aiello et al. Citation2000, van der Made and van der Made Citation1993).
Immobilization and principle of the measurements
Prior to coating, Au electrode (AuE) surface was polished with alumina slurries on a microfiber cloth to obtain a mirror surface. It was then thoroughly rinsed with double-distilled water and sonicated first in absolute ethanol and then in double-distilled water for 10 min to remove adsorbed particles. The electrode was then cleaned by five successive cyclic voltammetric sweeps between − 1.0 V and + 1.0 V in the 0.1 M HCl.
To form SAM the clean gold electrode was immersed into 100 mM cysteamine solution for 15 h. At the end of this period the cysteamine-modified gold electrode (CMAuE) was rinsed with double-distilled water to remove unbounded molecules on the electrode surface. Co-immobilization of palladium complex and peroxidase was carried out as follows: 3 μL of the 4 mg/100 mL palladium complex solution (prepared in chloroform) was deposited on the cysteamine-modified gold electrode (CMAuE) and let to dry to form cysteamine–palladium complex-modified gold electrode (CPMAuE). Then 5 μl of 1.0 mg/mL peroxidise (HRP) solution was also deposited on the modified electrode and let to dry again to construct a cysteamine–palladium complex-peroxidase-modified gold electrode (CPPerMAuE) . Finally, the electrode was immersed in the 25% glutaraldehyde solution for 30 min at 4°C.
The principle of the measurements is based on monitoring increase in the oxidation potential of palladium complex (at + 0.47 V vs Ag/AgCl) using amperometric detection. Oxidation potential of palladium complex related to the enzymatic reaction of peroxidase in phosphate buffer (pH: 7.0; 50 mM) was determined by measuring cyclic voltammetry between + 0.1 and + 1.0 V potentials. Correlations between increase in biosensor response and peroxidase activity were monitored. All the measurements were carried out at 30°C using thermostatic reaction cells and phosphate buffer (50 mM, pH 7.0).
Results and discussion
Cyclic voltammetry characterization of the biosensor
To characterize the biosensor, some cyclic voltammetric measurements were done after each modified step. showed the cyclic voltammograms (CVs) of bare and modified electrodes in different steps in phosphate buffer (pH 7.0), with a scan rate of 100 mV/s. From the figure it is obvious that cyclic voltammograms of the bare gold electrode, after cysteamine deposition, and co-immobilization of palladium complex and peroxidase showed the same behavior in the absence of H2O2. Therefore, the cysteamine–palladium complex-peroxidase-modified gold electrode (CPPerMAuE) showed a different cyclic voltammogram after injection of H2O2 (100 μM) into the reaction medium. Upon the addition of the H2O2 to the electrochemical cell, the oxidation peak of the palladium complex (at +0.47 V) appears, related to a typical electron transfer between the H2O2 and the HRP molecule. According to , an obvious effect of the palladium complex was shown in the enzymatic process of HRP, that is, the complex can act as a conduction center which facilitates the transfer of electrons.
Figure 1. Cyclic voltammograms (CVs) of bare and modified biosensors in different steps in phosphate buffer (pH 7.0), with a scan rate of 50 mVs− 1 vs Ag/AgCl. a) bare gold electrode, b) Cysteamine-modified gold electrode (CMAuE), c) Cysteamine–palladium complex-peroxidase-modified gold electrode (CPaPeMAuE) in the absence of H2O2, d) Cysteamine–palladium complex-peroxidase-modified gold electrode (CPaPeMAuE) in the presence of H2O2 (100 μM).
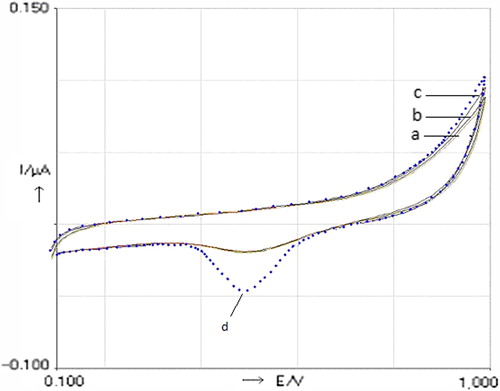
Optimization of bioactive layer and working condition of the biosensor
In order to optimize the components of bioactive layer of the biosensor effects of enzyme concentration, palladium complex amount, cysteamine concentration, and duration of SAM formation on the biosensor responses were investigated. From the experiments the best biosensor responses were obtained using 0.5 mgmL− 1 enzyme, 100 mM cysteamine, 40 mgmL− 1 palladium complex, and 15 h duration of cysteamine.
To detect the effect of pH on the electrocatalytical reduction of H2O2, the modified biosensor was studied under a constant concentration (100 μM) of H2O2 in different pH values (5.0–8.0) by amperometric measurements at an applied potential of +0.47 V. From the results, the current responses increased up to pH 7.0. Above this pH value, decreases in current responses were obtained. Therefore, pH 7.0 was selected to be the working buffer. The same measurements were done to obtain the optimum working temperature of the modified biosensor using different temperature values (15–40°C). Current responses obtained from the measurements increased up to 30°C. Further increase in temperature will lead to a decrease in the current response because of the partial denaturation of the enzyme. Considering both the lifetime and response characteristics of the enzyme, 30°C was employed as the optimum temperature to detect H2O2..
Electrochemical response to H2O2
To investigate chronoamperometric response of the modified biosensor, different concentrations of H2O2 (25, 10, 5, and 2.5 μM) were prepared and used in phosphate buffer (pH 7.0) at an applied potential of +0.47 mV. shows the chronoamperometric results of modified peroxidase biosensor after H2O2 injections with different concentrations. Injections were made from high to low concentrations of H2O2 to examine the concentration effect on the biosensor response and also to test whether there is any accumulation of high concentration of the substrate on the surface of the biosensor. There was an expected result in the experiments because the current increased with increasing H2O2 concentration. It can be said that the response of the biosensor is related to the concentration of H2O2 as a result of the enzymatic reaction.
Figure 2. Chronoamperometric results of modified peroxidase biosensor after H2O2 injections with different concentrations at +0.47 V potential vs Ag/AgCl.
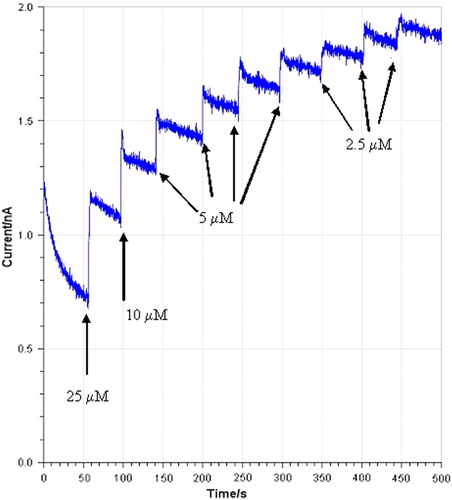
The amperometric measurement of H2O2 by the (CPPerMAuE) biosensor has been investigated, shows the response current of the successive addition of H2O2 from 2.5 to 150 μM. Calibration curve of the response current of the biosensor to H2O2 concentration is shown in . As expected, the response of the biosensor to H2O2 was linear in the range from 2.5 μM to 150 μM with a correlation coefficient (R) of 0.9908. The biosensor has a good detection limit of 1.2 μM (S/N = 3), and a short response time within 5 s.
Repeatability and stability of the H2O2 biosensor
When the modified biosensor was repeated for 15 successive measurements at 50 μM concentration of H2O2, the average value, standard deviation (± SD), and coefficient of variant (CV %) were calculated to be 49.85 μM, ± 1.09, and 2.2%, respectively. The storage stability of the biosensor was investigated by amperometric measurements in the presence of 50 μM H2O2 periodically. When not in use, it was stored under dry conditions at 4°C in a refrigerator. The biosensor lost only 17% of the initial response after storage for more than 1 month.
Selectivity of the H2O2 biosensor
To investigate the effect of some interferences toward H2O2 detection some experiments were also carried out by the modified biosensor. For this purpose, some interference substances such as glucose, ethanol, acetic acid, L-ascorbic acid, and uric acid were used in the amperometric measurements. The current ratios were calculated by reading the current of the biosensor in the assay solution containing 50 μM H2O2 and interfering substance and comparing it with the current from the biosensor in the same assay solution containing only 50 μM H2O2. In the experiments, the five tested substances did not interfere significantly with the modified biosensor. It is known that ascorbic acid (AA) is one of the chemical species in physiological fluid and is commonly oxidized at + 0.3 V vs Ag/AgCl. Therefore, no electrochemical response occurred at working potential (+ 0.47 V). This result increased the selectivity of the modified biosensor.
Conclusion
In this paper, a new peroxidase biosensor modified with cysteamine and a new palladium complex has been developed. From the cyclic, voltammetric, and chronoamperometric measurements, it can be said that palladium complex serves to be an efficient mediatoric system between peroxidase and gold electrode. The modified biosensor exhibited a fast amperometric response to H2O2 without any interference effects.
Declaration of interest
The authors report no declarations of interest. The authors alone are responsible for the content and writing of the paper.
References
- Aiello I, Crispini A, Ghedini M, La Deda M, Barigelletti F. 2000. Synthesis and characterization of a homologous series of mononuclear palladium complexes containing different cyclometalated ligands. Inorg Chim Acta. 308:121.
- Bartlett PN, Birkin PR, Wang JH, Palmisano F, Benedetto GD. 1998. An enzyme switch employing direct electrochemical communucitaion between horseradish peroxidase and a poly(aniline) film. Anal Chem. 70:3685.
- Brestovisky A, KirowaEisner E, Osteryoung J. 1983. Direct and titrimetric determination of hydrogen peroxide by reverse pulse polarography. Anal Chem. 65:523.
- Ferapontova E, Schmengler K, Börchers T, Ruzgas T, Gorton L. 2002. Effect of cysteine mutations on direct electron transfer of horseradish peroxidase on gold. Biosens Bioelectron. 17:953.
- Garguilo MG, Huynh N, Proctor A, Michael AC. 1993. Amperometric sensors for peroxide, choline, and acetylcholine based on electron transfer between horseradish peroxidase and a redox polymer. Anal Chem. 65:523.
- Gaspar S, Zimmermann H, Gazaryan I, Csöregi E, Schuhmann W. 2001. Hydrogen peroxide biosensors based on direct electron transfer from plant peroxidases immobilized on self-assembled thiol-monolayer modified gold electrodes. Electroanal. 13:284.
- Gorton L, Lindgren A, Larsson T, Munteanu FD, Ruzgas T, Gazaryan I. 1999. Direct electron transfer between heme-containing enzymes and electrodes as basis for third generation biosensors. Anal Chim Acta. 400:91.
- Grigor D, Maicaneanu A, Walcarius A. 2010. Iron-enriched natural zeolite modified carbon paste electrode for H2O2 detection. Electrochim Acta. 55:4050.
- Günay ME, Gümüşada R, Özdemir N, Dinçer M, Çetinkaya B. 2009. Synthesis, X-ray structures, and catalytic activities of (κ2-C,N)-palladacycles bearing imidazol-2-ylidenes. J Organomet Chem. 694:2343.
- Hanaoka S, Lin JM, Yamada M. 2001. Chemiluminescent flow sensor for H2O2 based on the decomposition of H2O2 catalyzed by cobalt(II)-ethanolamine complex immobilized on resin. Anal Chim Acta. 426:57.
- Heller A. 1990. Electrical wiring of redox enzymes. Acc Chem Res. 23:128.
- Hong J, Dai Z. 2009. Amperometric biosensor for hydrogen peroxide and nitrite based on hemoglobin immobilized on one-dimensional gold nanoparticle. Sens Actuators B Chem. 140:222.
- Li J, Tan SN, Ge HL. 1996. Silica sol–gel immobilized amperometric biosensor for hydrogen peroxide. Anal Chim Acta. 335:137.
- Mala Ekanayake EMI, Preethichandra DMG, Kaneto K. 2008. Bi-functional amperometric biosensor for low concentration hydrogen peroxide measurements using polypyrrole immobilizing matrix. Sens Act B Chem. 132:166.
- Matsubara C, Kawamoto N, Takamura K. 1992. Oxo[5,10,15,20-tetra (4-pyridyl) porphyrinato] titanium (IV): an ultra-high sensitivity spectrophotometric reagent for hydrogen peroxide. Analyst. 117:1781.
- Nakashima K, Maki K, Kawaguchi S, Akiyama S, Tsukamoto Y, Imai K. 1991. Peroxyoxalate chemiluminescence assay of hydrogen peroxide and glucose using 2,4,6,8-tetrathiomorpholinopyrimido[5,4-d]pyrimidine as a fluorescent component. Anal Sci. 7:709.
- Razola SS, Ruiz BL, Diez NM, Mark Jr, J-M, Kauffmann HB. 2002. Hydrogen peroxide sensitive amperometric biosensor based on horseradish peroxidase entrapped in a polypyrrole electrode. Biosens Bioelectron. 17:921.
- S Cosnier. 1999. Biomolecule immobilization on electrode surfaces by entrapment or attachment to electrochemically polymerized films. A review. Biosens Bioelectron. 14:443.
- Sellers RM. 1980. Spectropohotometric determination of hydrogen peroxide using potassium titanium (IV) oxalate. Analyst. 105:950.
- Şenel M, Çevik E, Abasıyanık MF. 2010. Amperometric hydrogen peroxide biosensor based on covalent immobilization of horseradish peroxidase on ferrocene containing polymeric mediator. Sens Act B Chem. 45:444.
- van der Made AW, van der Made RH. 1993. A convenient procedure for. Bromomethylation of aromatic compounds. Selective mono-, bis-, or. Trisbromomethylation. J Org Chem. 58:1262.
- Vreeke M, Maidan R, Heller A. 1992. Hydrogen peroxide and betanicotinamide adenine dinucleotide sensing amperometric electrodes based on electrical connection of horseradish peroxidase redox centers to electrodes, through a 3-dimensional electron relaying polymer network. Anal Chem. 64:3084.
- Wang BQ, Zhang JZ, Cheng GJ, Dong SJ. 2000. Amperometric enzyme electrode for the determination of hydrogen peroxide based on sol–gel/hydrogel composite film. Anal Chim Acta. 407:111.
- Wang J, Lin Y, Chen L. 1993. Organic-phase biosensors for assays of pharmaceutical products. Analyst. 118:277.
- Wang J, Wang L, Di J, Tu Y. 2009. Electrodeposition of gold nanoparticles on indium/tin oxide electrode for fabrication of a disposable hydrogen peroxide biosensor. Talanta. 77:1454.
- Xiao Y, Ju HX, Chen HY. 1999. A reagentless hydrogen peroxide sensor based on incorporation of horseradish peroxidase in poly(thionine) film on a monolayer modified electrode. Anal Chim Acta. 391:299.
- Yan L, Huck WTS, Whitesides GM. 2004. Self-Assembled Monolayers (SAMs) and Synthesis of Planar Micro- and Nanostructures. J Macromol Sci. Part C: Polymer Reviews 44:175.
- Zhang J, Oyama M. 2005. Gold nanoparticle-attached ITO as a biocompatible matrix for myoglobin immobilization: direct electrochemistry and catalysis to hydrogen peroxide. J Electroanal Chem. 577:273.