Abstract
Context: The conjugation of silver nanoparticles (AgNPs) with biomolecules such as oligosaccharides, DNA, proteins has attracted great attention of scientists recently. Objective: In this study, lysozyme–AgNP conjugates were evaluated for its synergic antimicrobial effect. Materials and methods: AgNPs were synthesized and characterized using UV-Visible, X-ray diffraction analysis (XRD) and atomic force microscopy (AFM). AgNP (0–1 mM) was interacted with lysozyme for multi-spectrophotometric studies. Lysozyme was immobilized on AgNP at different ratios and the resulting nano-bio-conjugate was tested against Escherichia coli for potent synergic antibacterial effects. Results and discussion: A surface plasmon peak at 420 nm confirmed the presence of AgNPs and spherical to oval-shaped AgNPs were observed by AFM. The particle size was calculated to be 25 nm by XRD analysis. The maximum immobilization efficiency (98%) was achieved at 0.01:1 ratio of enzyme:AgNP. UV–Visible and fluorescence spectral studies revealed the binding of AgNPs to lysozyme by the formation of ground-state complex and the binding parameters were calculated. Circular dichroism studies confirmed decrease by 11% in the α-helical and 29.32% in β-sheets of lysozyme upon AgNP interaction. FTIR spectra revealed the binding of AgNP through thiol (–SH) linkages of lysozyme. Our results showed that the antimicrobial activity of lysozyme-AgNP conjugate was enhanced up to 86% decrease in the cell growth. Conclusion: In summary, the immobilization of lysozyme on AgNP has yielded a nano-bio-conjugate with synergistic antibacterial properties.
Introduction
Lysozyme was chosen as a conjugating agent with AgNP as it holds bactericidal properties. Lysozyme is a monomeric globular protein comprising 129 amino acids which is capable of degrading bacterial membrane structure by catalyzing the hydrolysis of the bond (1,4-beta-linkages) between N-acetylmuramic acid and N-acetyl-D-glucosamine residues in peptidoglycans (Imoto et al. Citation1972, Pellegrini et al. Citation1997, Pellegrini et al. Citation2000). Lysosome, the cellular component secreting lysozyme, contains 50–60 hydrolases that constitute the cellular site for bulk macromolecule degradation and functions to mediate several processes in cell feeding and antimicrobial defence (Bang et al. Citation2011, Coleman et al. Citation1970, Yoon et al. Citation2009). Moreover, lysozyme has applications in pharmaceutical products and food because of its antimicrobial characteristics (Ibrahim et al. Citation2001, Goyal et al. Citation2009, Yoon et al. Citation2009).
The use of nano-sized materials offers various advantages owing to their small size and physical properties. Nanoparticles (NPs) and nanoparticle-conjugates have gained interest in the recent years for many biomedical applications (Murphy et al. Citation2005). They are being considered as drugs of their own with much interest as the biocidal activities of Ag have been well documented (Ruparelia et al. Citation2008). In the recently report, AuNPs enhanced the biocidal activity of growing and resting Escherichia coli cells when biomolecules were conjugated to them (Santos et al. Citation2012). NP–biomolecule interaction studies have contributed an understanding the reaction of binding kinetics, binding energy and structural changes of the biomolecule through various analytical techniques (Iseult and Dawson Citation2008, Aubin-Tam and Hamad-Schifferli Citation2008, Ernest et al. Citation2013a). Though many attempts were done to improve enzyme stability via enzyme immobilization, enzyme modification, protein engineering, NPs are used in the recent years for enzyme immobilization. It is either done by attachment or by incorporation of enzyme molecules via simple adsorption, covalent attachment, electrostatic binding or encapsulation using protein cages over NPs which has gained interest among researchers (Pascoal et al. Citation2011, Karim et al. Citation2012). Magnetite NPs are widely used for immobilization (Namdeo and Bajpai Citation2009, Sahoo et al. Citation2012, Zhang et al. Citation2011), since the recovery process of enzyme is easier.
Silver is considered the best conductor among metals (Kraus Citation1922). Silver nanoparticles (AgNPs) have also been proved for enhancing the catalytic activity of enzymes upon immobilization. The catalytic activity of α-amylase was increased in the presence of AgNPs when immobilized (Ernest et al. Citation2012). The oxidative coupling of thiols to disulfides was catalysed by PVP-capped AgNPs (Yan et al. Citation2009). In the last decade, the biomaterial applications of AgNPs have increased in numerous fields (Maynard and Michelson Citation2006, CitationGuerrini et al. 2009, Krajewski et al. Citation2013, Ravindran et al. Citation2013, Wang et al. Citation2008, Kim et al. Citation2007, Phoenix and Drexler Citation2004). AgNPs have been used as effective antimicrobial agent against many species of pathogenic organisms (Perelshtein et al. Citation2008, Samundeeswari et al. Citation2012, Tripathi et al. Citation2009, Vimalanathan et al. Citation2012).
Till now, independent action of either lysozyme or AgNPs on the bactericidal effect has been in focus of research but this is the first of its kind to immobilize lysozyme on AgNPs and to study its synergic antibacterial effect. In this present study, we have also attempted to study the secondary structure changes in enzyme upon AgNP by multi-spectrophotometric methods, as there were no systematic procedures to draw specific conclusions to obtain an insight into the specificity of interaction. Antibactericidal test was done to compare the activity of free and immobilized lysozyme.
Materials and methods
Chemicals
Silver nitrate was purchased from Merck, India. Sodium borohydride and tri-sodium citrate di-hydrate were obtained from SD-Fine Chemicals, India. Lysozyme (hen egg-white) was obtained from Sigma Aldrich, USA. For protein studies, 0.1 M phosphate buffer (pH 7.2) was used unless otherwise indicated.
Synthesis of AgNPs
AgNPs were prepared according to Creighton's method with minor modifications. Briefly, 30 ml of 2 mM ice-chilled solution sodium borohydride was mixed with 2 ml of 1% tri-sodium citrate while stirring for 5 min. Ten millilitres of 1 mM silver nitrate solution was added drop by drop under dark conditions without stirring. Pale yellow colour marks the end of the reaction and confirms the formation of particles. The solution was left overnight and centrifuged at 15,000 rpm for 30 min. The pellet was resuspended in distilled water. This procedure was done twice and the final pellet was suspended in distilled water and lyophilized. The required concentration was used for the experiments.
Immobilization of lysozyme with AgNPs
For the immobilization of lysozyme, 1 mg of AgNP was used for this study. The 1 mg of AgNPs was treated with solutions of lysozyme of 0.01, 0.02, 0.03, 0.1, 0.2, 0.3, 1, 2 and 3 mg, respectively, in 0.1 M sodium phosphate buffer (pH 6.0) for a reaction time of 30 min.
Efficiency of immobilization
The immobilization efficiency of the lysozyme on AgNP was calculated by measuring protein concentration in the free enzyme fraction and the residual fraction after immobilization. The protein concentration in the reaction before and after immobilization was determined using Bradford method (Bradford Citation1976).
Effect of AgNP on the structure of lysozyme
UV–visible spectral studies
UV–visible spectroscopic measurements were performed on a Double-beam spectrophotometer (Systronics 2201) with 10-mm path length quartz cuvettes for analysis. The absorption spectra for the free lysozyme and lysozyme interacted with AgNP were recorded in the range of 250–350 nm. The amylase concentration was constant and AgNP concentration was varied as mentioned in Section 2.2.
Fluorescence spectroscopy
Intrinsic fluorescence intensity of the protein (Trp) residues by AgNPs was carried out at an excitation wavelength (280 nm) by ELICO Spectrofluorimeter. The widths of the excitation and the emission slits were set to 10 nm. The fluorescence spectra of free amylase and AgNP-amylase (0, 0.2, 0.4, 0.6, 0.8 and 1 mM) were recorded. All experiments were done in triplicates to ensure repeatability. The experiments were performed in phosphate buffer (pH 7.4).
FT-IR spectroscopy
FT-IR spectra were recorded on PerkinElmer SpectrumOne spectrometer, operated at a resolution of 1.0 cm− 1 with KBr and mylar beam splitter. Pure amylase and AgNP-amylase were lyophilized and the spectra were read between 4500 and 400 cm− 1 with a resolution of 1 cm− 1. The spectra of the samples were recorded thrice and averaged to ensure reproducibility. All the spectral measurements were performed at 25 ± 3°C.
Circular dichroism spectroscopy
Circular dichroism (CD) spectra of native lysozyme and lysozyme–AgNP were recorded using JASCO J-715 CD spectrophotometer. Lysozyme concentration was maintained as 0.5 mg/ml and various concentration of AgNP (0–1 mM) was added. For measurements in the far-UV region (200–260 nm), a quartz cell with a path length of 1 mm was used for sampling. Three scans with a scan speed of 20 nm/min were performed and averaged. Samples were maintained at 25°C during the analysis. A spectrum of blank solution was subtracted from the spectra of AgNP–lysozyme samples. The secondary structure per cent was determined using the software JASCO Corp., J-715 manufacturer (Tokyo, Japan).
Antimicrobial activity
The bacterial culture E. coli 2809 (NCIM cultures) obtained from NCL, Pune, India was used for the study. An antibacterial test was done to compare the bactericidal activities of free lysozyme and immobilized lysozyme. The test organism, E. coli cells (2809 NCIM), was grown till the turbidity reached 0.1 (optical density) at 37°C, the cells were diluted to105 cells/ml. The E. coli cells with lysozyme:AgNP (0.01:1 mg/mg) was diluted in 900 μl of buffer and was spread over a LB medium. The antibacterial activities were compared by calculating the number of colonies after 16 h of bacterial growth.
Statistical analysis
All data presented in this study are mean ±SE. The experiments were carried out in triplicates and measured simultaneously to ensure reproducibility. These data were validated by t test at p < 0.05. Two-way ANOVA followed by Bonferonni post-test were carried out to analyse the statistical significance of treatment and concentration exposure dependence for antibacterial studies. The differences were considered significant if p < 0.05 and the data were analysed using GraphPad Prism 5.
Results and discussion
Synthesis and characterization of AgNPs
The reduction of silver nitrate by sodium borohydride resulted in a yellow colour colloidal solution of AgNPs exhibiting an intense surface plasmon absorbance band at 420 nm (). A good symmetry of the curve around the peak shows the homogenous distribution of the AgNPs. From the XRD analysis, the corresponding facets of silver such as [101], [111], [200], [220] and [311] with the Bragg's reflection at 32.6°, 38.1°, 44.2°, 64.4° and 77.3°, respectively, were exhibited according to the (JCPDS no. 04-0783) standards as it represents the face-centred cubic (fcc) structure of the NPs (). According to the Debye–Scherrer equation, the size of the particles was calculated to be 22.53 nm. The shape of the silver NPs was found to be predominantly spherical, whereas, few oval-shaped particles were also seen which showed a uniform distribution () by atomic force microscopy.
Figure 1. Characterization of synthesized AgNPs. (a) UV–Vis spectrum of AgNPs (b) XRD pattern of AgNPs (c) AFM image of AgNPs.
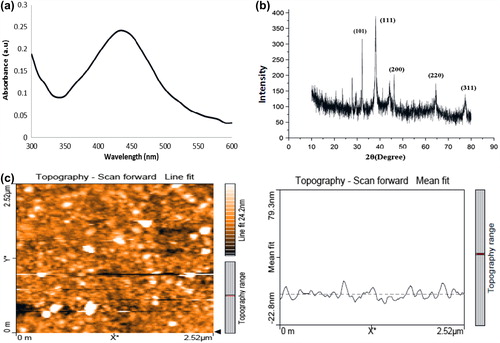
Immobilization efficiency of lysozyme onto AgNPs
The immobilization efficiency of lysozyme onto AgNPs was analysed after a reaction time of 30 min. To find the maximum efficiency of immobilization, different ratios (mg/mg) of enzyme and AgNP were checked and found to have 98% immobilization () at the interaction concentration of 1:100 (lysozyme:AgNP). The immobilization efficiency of the lysozyme on AgNP was calculated by measuring protein concentration in the supernatant and the pellet obtained after centrifugation after immobilization. The efficiency of immobilization of lysozyme on AgNPs was maximum at the concentration of 1:100 (lysozyme:AgNP). At a lower concentration of lysozyme, adsorption was found to be more efficient on the surface of AgNPs. This suggests that the particle size of the adsorbent has the influence on the immobilization efficiency of enzyme retention onto it. A similar study on conjugating lysozyme on rutile and anatase TiO2 nanopowders had reported the maximum efficiency of immobilization at the lowest concentration of the enzyme on anatase TiO2 particles which claimed that the total pore volume of anatase was higher when compared to the rutile type (Eby et al. Citation2009, Bang et al. Citation2011).
Effect of AgNP on the structure of lysozyme
UV–visible spectral studies
UV–visible spectroscopy is a powerful tool to study the changes in structural conformation of proteins (Skujins and Varian Citation1986). Spectral change of lysozyme has been monitored upon interaction of different concentrations of AgNPs. Lysozyme showed the maximum UV absorbance at 280 nm. Upon the addition of AgNPs (0–1 mM), the intensity of the absorbance of lysozyme was found to be increasing (). The absorbance of lysozyme was recorded at λmax of 280 nm, whereas, on addition of AgNPs, a plasmon shift occurred as AgNP concentration increases as seen in . The intensity at the wavelength of 280 nm increases at 0.6 mM AgNP significantly with a blue shift of 8 nm. The absorption band of lysozyme increased with the concentration of AgNPs. This is due to the fact that the secondary structure of lysozyme was found to be disturbed by the interaction with NPs and hence the exposure of the heterocyclic aromatic rings resulted in the increase of the UV absorbance (Hidaka et al. Citation1997, Mandal et al. Citation2010). describes the absorption intensity plot between AgNPs and lysozyme. The binding of AgNPs to lysozyme was valid as the plot of concentration of AgNP versus 1/(A− A0) yields a linear line with a R2 value of 0.9482.
Fluorescence spectroscopy
Fluorescence quenching is defined as any process that tends to decrease the fluorescence intensity of a sample due to the molecular interactions that results in quenching. These include excited state reactions, molecular rearrangements, energy transfer, ground-state complex formation and collisional quenching (Bhogale et al. Citation2012, Mariam et al. Citation2011, Kathiravan and Renganathan Citation2008). Fluorescence was measured by excitation at 280 nm and emission at 310–400 nm. shows the fluorescence emission spectra of lysozyme and AgNPs interacted lysozyme. It was found that as the AgNPs concentration increases, the emission intensity of lysozyme was found to be gradually decreasing with the peak shift of about 5 nm. The fluorescence quenching data at different concentration of NPs was obtained using Stern–Volmer equation [Citation23]:
Figure 4. (a) Fluorescence spectra intensity quenching as indicated by (b) Stern–Volmer plots of NP quenching, (c) energy transfer efficiency plot and (d) double-lograthmic plots representing the binding constant (K) and number of binding sites (n).
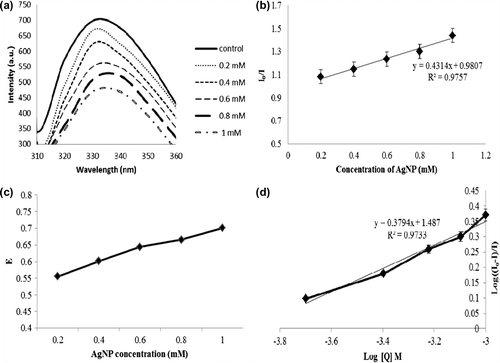
where I0 and I represent the steady-state fluorescence intensities in the absence and presence of AgNPs, respectively. Ksv is the Stern–Volmer quenching constant, [Q] is the NPs concentration (Molar) and Kq is the quenching rate constant, τ0 average life time of the molecule without any quencher. The slope obtained from the Stern–Volmer plot () represents the Ksv, [as the fuorescence lifetime of a biopolymer is 10 − 8 s (Kathiravan and Renganathan Citation2008, Lakowicz et al, Citation1980)] and was found to be 1.03 × 1013 M− 1s− 1, respectively.
A plot of the values for energy transfer efficiency was plotted according to the equation:
where I and I0 are the fluorescence intensities of the protein in the presence and absence of AgNPs, respectively. The line was linear and increasing with increase in AgNP concentration. This denotes that the energy has been transferred from the valence band of Ag to Trp residues by quenching the fluorescence intensity of the residues (). It is seen that ‘E’ value steadily increases with increase in AgNP concentration. represents the double-logarithmic plot from which the binding parameters for lysozyme–AgNP were calculated () according to the double-logarithmic plot equation:
where I and I0 are the fluorescence intensities of lysozyme in the presence and absence of AgNPs, respectively, K represents the binding constant, n represents the number of binding sites and Q represents the AgNP concentration.
CD spectroscopy
CD studies are considered significant in studying the secondary structure of proteins (Berova et al. Citation2000). The spectra profile of native lysozyme and AgNP–lysozyme at different concentrations between 200 and 260 nm were recorded and shown in . The dichroic band at 208 nm is seen clearly which is a characteristic band pattern of α-helical structure. From CD data it is apparent that the spectral profiles of AgNP–lysozyme are similar to those of the protein in its native form. Upon interaction of different concentration AgNPs with the enzyme, a progressive reduction in intensity of the diagnostic bands at 208 nm was seen indicating minor changes that the protein conformation has slightly changed compared with its free state. There was a decrease by 11% in the α-helical and 29.32% in β-sheets content, whereas 1.1% increase and 13.93% increase in turns and random structures () upon AgNP interaction at the maximum concentration (1 mM) as computed by the JASCO software from the manufacturer (JASCO Corp.). Deformation in secondary structure leads to change in the protein's native 3D structure, wherein, the function of the protein could also be altered. Far-UV CD spectra revealed the change in the amylase–AgNP complex having deformation leading to structural and functional change which might be considered significant in catalysis (Zolghadri et al. Citation2010, Karim et al. Citation2012). In similar systems, the conformation of protein adsorbed onto solid surfaces is almost the same as in bulk (Ernest et al. Citation2013b). These results suggest that the conformation of the enzyme has not changed in its bound state.
FTIR spectral studies
FTIR spectroscopy is proved to be the valuable method for monitoring the secondary structural changes in lysozyme before and after interaction with AgNPs. The most spectral regions corresponding to the secondary structures of the protein are Amide A at 3300 cm− 1 due to NH stretching, Amide I at 1600–1690 − 1 due to C = O stretching and Amide II at 1480–1575 cm− 1 due to CN stretching and NH bending. Therefore, the shifts in the intensity of these regions denote the secondary structural confirmation changes (). It is very interesting to note that upon AgNP interaction there were no significant shifts of the FTIR bands in residues. Among the various bands of lysozyme, the absorbance intensity of the amide II band in the region 1500–1600 cm− 1 (C–N stretch coupled with N–H bending mode) (Sabatino et al. Citation2007), but it is not very much sensitive to the conformation of the lysozyme. The amide I band in the region 1600–1700 cm− 1 possess a tight relationship with the secondary structure of protein. Different regions of the amide I band were found to be contributed with different secondary structural elements: 1600–1639 cm− 1 by beta sheet, 1640–1650 cm− 1 by gamma-random coil, 1651–1660 cm− 1 by alpha-helix and 1661–1700 cm− 1 by t-turns (Johnston et al. Citation1996).
In , the FTIR spectra of the native lysozyme and lysozyme upon AgNP (1 mM) interaction is shown. As compared with the native lysozyme, the band of lysozyme after adsorption to NPs has showed the shifts in peak position (). This has indicated the occurrence of the distinct conformational change in lysozyme during its adsorption to the AgNPs. Amide I band of native lysozyme was found to be shifted from 1658 to 1641 cm− 1 that corresponds to the structural change or interaction of amino acids participating in the α-helical structure of the protein. This is in corroboration with the CD results which denotes 11% decrease in the α-helical content. Same way, the amide II band of the native lysozyme was shifted from 1535 to 1566 cm− 1.
Table I. Characteristic peak positions of protein bands of native lysozyme and peak shifts for lysozyme–AgNP interaction.
Table II. Binding parameters (Stern-Volmer constant (Ksv), binding constant (K) and no. of binding sites (n)) of AgNPs interacted with lysozyme.
The thiol linkage present at 702 cm− 1 in the native lysozyme spectra was completely diminished in the AgNP–lysozyme sample and shifted to 860 cm− 1. The FTIR data showed the absence of the –SH in all the AgNP–protein spectra confirming that the mode of binding of AgNPs was through the thiol group present in amino acids containing –SH groups. These functional groups could be protonated, enhancing the binding of those residues to AgNP surface through ligand exchange reactions (Ravindran et al. Citation2012). These distinct changes in the spectral regions denote the conformational changes in the lysozyme molecules during its interaction with AgNPs. There were no major shifts in protein bands in amide I, II and III bands upon AgNP interaction were recorded and are summarized in .
Antibacterial studies
E. coli was used as the test organism to evaluate the antimicrobial effect of the free and immobilized lysozyme. This is due to the fact that the information obtained from the organism was found to be relevant in understanding the serious and complicated bacterial infections of man (Doyle Citation1991). As the concentration of lysozyme increased, bacterial growth inhibition was seen increasing with the synergistic effect of AgNP and lysozyme. Cell viability of E. coli was found to be 49.15% at the lowest lysozyme:AgNP ratio (0.01:1) whereas only 14% cells were viable at their maximum interaction (3:1) ratio (). In a similar study using TiO2 nanoparticles, a ratio of 1:10 (lysozyme:NP) had a cell mortality of 60% (Bang et al. Citation2011). The results from the antibacterial test at the ratio of 1:100 of lysozyme and AgNP suggested higher bactericidal properties than free lysozyme. It is worthwhile to note that the synergic effects of lysozyme and AgNPs had brought about the effective killing of the microbe (Das et al. Citation2009, Eby et al. Citation2009, Franca de Oliveira et al. Citation2012). In the absence of extensive studies on this synergistic effect, in this current study we report 86% decrease in the cell growth upon lysozyme immobilization on AgNP. The enhanced synergistic effect of AgNP and lysozyme against pathogenic bacteria could be potentially applied in the development of new therapeutic agents.
Conclusion
In summary, the application of lysozyme immobilized AgNP as a potent antibacterial agent and its interaction study has been explored in detail. AgNP of uniform distribution, face-centred cubic crystalline pattern and spherical to oval shape was obtained. UV and fluorescence spectroscopic studies showed the complex formation between lysozyme and AgNP that lead to the conformational changes. The conformation of protein inferred by CD does not significantly change upon binding onto AgNPs. FTIR has revealed the interaction was primarily due to the thiol linkages (–SH) present in the enzyme. Due to the immobilization of lysozyme onto AgNP increased the antibacterial activity against E. coli cells synergistically which was proved by the cell viability tests.
Declaration of interest
The authors report no declarations of interest. The authors alone are responsible for the content and writing of the paper.
References
- Aubin-Tam M-E, Hamad-Schifferli K. 2008. Structure and function of nanoparticle–protein conjugates. Biomed Mater. 3:034001.
- Bang SH, Jang A, Yoon J, Kim P, Kim JS, Kim YH, Min J. 2011. Evaluation of whole lysosomal enzymes directly immobilized on titanium (IV) oxide used in the development of antimicrobial agents. Enzyme Microb Technol. 49:260–265.
- Berova N, Nakanishi K, Woody RW. 2000. Circular Dichroism: Principles and Applications. New York: Wiley-VCH.
- Bhogale A, Patel N, Sarpotdar P, Mariam J, Dongre P, Miotello A, Kothari D. 2012. Systematic investigation on the interaction of bovine serum albumin with ZnO nanoparticles using fluorescence spectroscopy. Colloids Surf B Biointerfaces. 102:257–264.
- Bradford MM. 1976. A rapid and sensitive method for the quantitation of microgram quantities of protein utilizing the principle of protein-dye binding. Anal Biochem. 72:248–254.
- Coleman S, van de Rijn I, Bleiweis A. 1970. Lysis of grouped and ungrouped streptococci by lysozyme. Infect Immun. 2:563–569.
- Das R, Jagannathan R, Sharan C, Kumar U, Poddar P. 2009. Mechanistic study of surface functionalization of enzyme lysozyme synthesized Ag and Au nanoparticles using surface enhanced Raman spectroscopy. J Phys Chem C113:21493–21500.
- Doyle M. 1991. Escherichia coli O157:H7 and its significance in foods. Int J Food Microbiol. 12:289–301.
- Eby DM, Luckarift HR, Johnson GR. 2009. Hybrid antimicrobial enzyme and silver nanoparticle coatings for medical instruments. ACS Appl Mater Interfaces. 1:1553–1560.
- Ernest V, Mukherjee A, Chandrasekaran N. 2013a. Interaction of Colloidal Ag Nanoparticles with Bovine Serum Albumin. In: Uversky VN, Kretsinger RH, Permyakov EA, Eds. Encyclopedia of Metalloproteins. New York: Springer.
- Ernest V, Nirmala J, Sekar G, Mukherjee A, Chandrasekaran N. 2013b. Biophysical investigation of α-Amylase conjugated silver nanoparticles proves structural changes besides increasing its enzyme activity. J Bionanosci. 7:1–5.
- Ernest V, Shiny PJ, Mukherjee A, Chandrasekaran N. 2012. Silver nanoparticles: a potential nanocatalyst for the rapid degradation of starch hydrolysis by α-amylase. Carbohydr Res. 352:60–64.
- Franca de Oliveira L, de Almeida Goncalves K, Boreli FH, Kobarg J, Cardoso MB. 2012. Mechanism of interaction between colloids and bacteria as evidenced by tailored silica-lysozyme composites. J Mater Chem. 22:22851–22858.
- Goyal MK, Roy I, Banerjee UC, Sharma VK, Bansal AK. 2009. Role of benzyl alcohol in the prevention of heat-induced aggregation and inactivation of hen egg white lysozyme. Eur J Pharm Biopharm71:367–376.
- Guerrini L, Garcia-Ramos JV, Domingo Cn, Sanchez-Cortes S (2009). Nanosensors based on viologen functionalized silver nanoparticles: few molecules surface-enhanced Raman spectroscopy detection of polycyclic aromatic hydrocarbons in interparticle hot spots. Anal Chem. 81:1418–1425.
- Hidaka H, Horikoshi S, Ajisaka K, Zhao J, Serpone N. 1997. Fate of amino acids upon exposure to aqueous titania irradiated with UV-A and UV-B radiation Photocatalyzed formation of NH3, NO3−, and CO2. J Photochem Photobiol A Chem. 108:197–205.
- Ibrahim HR, Matsuzaki T, Aoki T. 2001. Genetic evidence that antibacterial activity of lysozyme is independent of its catalytic function. FEBS Lett. 506:27–32.
- Imoto T, Johnson LN, North ACT, Phillips DC, Rupley JA. 1972. Vertebrate Lysozymes. In Boyer PB, Ed. The Enzymes. Academic Press, New York.
- Iseult L, Dawson KA. 2008. Protein-nanoparticle interactions. NanoToday. 3:40–47.
- Johnston C, Aochi Y, Sparks D, Page A, Helmke P, Loeppert R, et al. 1996. Fourier transform infrared and Raman spectroscopy. In: Methods of soil analysis. Part 3 - chemical methods. Madison: Amer Society of Agronomy; 269–321.
- Karim Z, Adnan R, Ansari MS. 2012. Low concentration of silver nanoparticles not only enhances the activity of horseradish peroxidase but alter the structure also. PLoS One. 7:e41422.
- Kathiravan A, Renganathan R. 2008. Interaction of colloidal TiO2 with bovine serum albumin: A fluorescence quenching study. Colloids Surf A Physicochem Eng Asp. 324:176–180.
- Kim JS, Kuk E, Yu KN, Kim JH, Park SJ, Lee HJ, et al. 2007. Antimicrobial effects of silver nanoparticles. Nanomedicine. 3:95–101.
- Krajewski S, Prucek R, Panacek A, Avci-Adali M, Nolte-Karayel A, Straub A, et al. 2013. Hemocompatibility evaluation of different silver nanoparticle concentrations employing a modified Chandler-loop in vitro assay on human blood. Acta Biomater. 9:7460–7468.
- Kraus CA. 1922. The constitution of metallic substances. J Am Chem Soc. 44:1216–1239.
- Lakowicz J, Freshwater G, Weber G. 1980. Nanosecond segmental mobilities of tryptophan residues in proteins observed by lifetime-resolved fluorescence anisotropies. Biophys J. 32:591–601.
- Mandal G, Bardhan M, Ganguly T. 2010. Interaction of bovine serum albumin and albumin-gold nanoconjugates with l-aspartic acid. A spectroscopic approach. Colloids Surf B Biointerfaces. 81:178–184.
- Mariam J, Dongre P, Kothari D. 2011. Study of interaction of silver nanoparticles with bovine serum albumin using fluorescence spectroscopy. J Fluoresc. 21:2193–2199.
- Maynard A, Michelson E. 2006. The nanotechnology consumer products inventory. Woodrow Wilson International Center for Scholars, Washington, DC, accessed March, 23.
- Murphy CJ, Sau TK, Gole AM, Orendorff CJ, Gao J, Gou L, et al. 2005. Anisotropic metal nanoparticles: synthesis, assembly, and optical applications. J Phys Chem B. 109:13857–13870.
- Namdeo M, Bajpai S. 2009. Immobilization of α-amylase onto cellulose-coated magnetite (CCM) nanoparticles and preliminary starch degradation study. J Mol Catal B Enzym. 59:134–139.
- Pascoal AM, Mitidieri S, Fernandes KF. 2011. Immobilisation of α- amylase from Aspergillus niger onto polyaniline. Food Bioprod Process. 89:300–306.
- Pellegrini A, Thomas U, Bramaz N, Klauser S, Hunziker P, Von Fellenberg R. 1997. Identification and isolation of a bactericidal domain in chicken egg white lysozyme. J Appl Microbiol. 82:372–378.
- Pellegrini A, Thomas U, Wild P, Schraner E, Von Fellenberg R. 2000. Effect of lysozyme or modified lysozyme fragments on DNA and RNA synthesis and membrane permeability of Escherichia coli. Microbiol Res. 155:69–77.
- Perelshtein I, Applerot G, Perkas N, Guibert G, Mikhailov S, Gedanken A. 2008. Sonochemical coating of silver nanoparticles on textile fabrics (nylon, polyester and cotton) and their antibacterial activity. Nanotechnology. 19:245705.
- Phoenix C, Drexler E. 2004. Safe exponential manufacturing. Nanotechnology. 15:869.
- Ravindran A, Chandran P, Khan SS. 2013. Biofunctionalized silver nanoparticles: advances and prospects. Colloids Surf B Biointerfaces. 105:342–352.
- Ravindran A, Chandrasekaran N, Mukherjee A. 2012. Studies on differential behavior of silver nanoparticles towards thiol containing amino acids. Curr Nanosci. 8:141–149.
- Ruparelia JP, Chatterjee AK, Duttagupta SP, Mukherji S. 2008. Strain specificity in antimicrobial activity of silver and copper nanoparticles. Acta Biomater. 4:707–716.
- Sabatino P, Casella L, Granata A, Iafisco M, Lesci IG, Monzani E, Roveri N. 2007. Synthetic chrysotile nanocrystals as a reference standard to investigate surface-induced serum albumin structural modifications. J Colloid Interface Sci. 314:389–397.
- Sahoo B, Sahu SK, Bhattacharya D, Dhara D, Pramanik P. 2012. A novel approach for efficient immobilization and stabilization of papain on magnetic gold nanocomposites. Colloids Surf B Biointerfaces. 101:280–289.
- Samundeeswari A, Dhas SP, Nirmala J, John SP, Mukherjee A, Chandrasekaran N. 2012. Biosynthesis of silver nanoparticles using actinobacterium Streptomyces albogriseolus and its antibacterial activity. Biotechnol Appl Biochem. 59:503–507.
- Santos M, Queiroz M, Baptista P. 2012. Enhancement of antibiotic effect via gold:silver-alloy nanoparticles. J Nanopart Res. 14:1–8.
- Skujins S, Varian A. 1986. Applications of UV-Visible Derivative Spectrophotometry. UV Instruments at work, Part2, 1, 1–50.
- Tripathi A, Chandrasekaran N, Raichur A, Mukherjee A. 2009. Antibacterial applications of silver nanoparticles synthesized by aqueous extract of Azadirachta indica (Neem) leaves. J Biomed Nanotechnol. 5:93–98.
- Vimalanathan AB, Ernest V, Arumugasamy K, Tyagi MG. 2012. Biosynthesis of silver nano-particles by the bacterium Micrococcus luteus. Int J Appl Biol Pharm Technol. 4:77–83.
- Wang AZ, Gu F, Zhang L, Chan JM, Radovic-Moreno A, Shaikh MR, Farokhzad OC. 2008. Biofunctionalized targeted nanoparticles for therapeutic applications. Expert Opin Biol Ther. 8:1063–1070.
- Yan J, Tao H, Zeng M, Tao J, Zhang S, Yan Z, et al. 2009. PVP-capped silver nanoparticles as catalyst for oxidative coupling of thiols to disulfides. Chin J Catal. 30:856–858.
- Yoon J, Park JM, Kim KJ, Kim YH, Min J. 2009. Antimicrobial activity of the cell organelles, lysosomes isolated from egg white. J Microbiol Biotechnol. 19:1364.
- Zhang X, Jiang W, Zhou Y, Xuan S, Peng C, Zong L, Gong X. 2011. Magnetic recyclable Ag catalysts with a hierarchical nanostructure. Nanotechnology. 22:375701.
- Zolghadri S, Saboury A, Amin E, Moosavi-Movahedi A. 2010. A spectroscopic study on the interaction between ferric oxide nanoparticles and human hemoglobin. J Iran Chem Soc. 7: 145–153.