Abstract
Calcium phosphates are one of the biomaterials that are used for bone regeneration. In this study, Hydroxyapatite (HAp) nanoparticles with chitosan gel filled with unrestricted somatic stem cells (USSCs) were used for healing calvarial bone in rat model. The healing effects of these injectable scaffolds with and without stem cells for bone regeneration were investigated by computed tomography (CT) analysis and pathology assays after 28 days of grafting. The results of CT analysis showing bone regeneration on the scaffolds, also the amounts of regenerated new bone for USSC scaffold was significantly greater than the scaffold without cell and untreated controls. Therefore, the combination of scaffold especially with USSC is considered as a useful method for bone regeneration.
Introduction
The healing of bone defects with large gaps is the problem of many patients. Using biological scaffolds or non-biodegradable synthetic compounds with unique properties have been able to repair small defects (CitationMonteiro et al. 2012, CitationHorwitz et al. 1999, CitationMeirelles et al. 2006, CitationBranski et al. 2009). Human umbilical cord blood is a rich source of hematopoietic stem cells for clinical application and may be one of the wide sources of stem cells with naive immune status (CitationKamolz et al. 2006, CitationWilson et al. 2011). Cord lining-mesenchymal stem cells express CD23, CD14, and low amounts of CD34 and CD35; they are not able to express endothelial marker CD31 and have greater expansion in vitro than Wharton's jelly-derived MSCs (CitationIshige et al. 2009). CD14 inhibits T cells. Wharton's jelly-derived MSCs do not express CD14 or CD23. Despite those descriptions, the cell markers of umbilical cord-derived MSCs still remain under consideration (CitationKita et al. 2010, CitationWu et al. 2007). One of the key factors of tissue engineering is to prepare a three-dimensional scaffold with suitable properties and also, high porosity, interconnected pores and so on. In natural tissues, cells are surrounded by extracellular matrix, which has a physical structural features ranging from nanometer scale to micrometer. To mimic the natural extra cellular matrix, many researches has been done based on fabricate nanostructured scaffold by different methods such as sol-gel (CitationWang 2003, CitationLiu et al. 2001, CitationWang et al. 2005). Chitosan (CS) displays a number of properties including biocompatibility, degradability, mucoadhesiveness, and an ability to promote wound healing letting CS sponges, films, gels, beads, etc. to be developed and used in various biomedical applications (CitationDi Martino et al. 2005, CitationKim et al. 2008, CitationJayakumar et al. 2010, CitationShi et al. 2006, CitationVenkatesan and Kim 2010, CitationChesnutt et al. 2009a, Citation2009b, CitationReves et al. 2009). Furthermore, CS can induce cell attachment and proliferation; predominantly due to structural similarity of CS polysaccharide backbone to glycosaminoglycans, the major component of the extra cellular matrix of bone (CitationKhor and Lim 2003, CitationZhang and Zhang 2001). Combination of two materials with different characteristics leading to composites with tailor-made properties is well known (CitationHuang et al. 2009). Hydroxyapatite [HAp, Ca10(PO4)6(OH)2] is a type of calcium phosphates, which has extensive applications in the healing of bone and tooth, due to biocompatibility and similar composition to natural bone (CitationSuchanek and Yoshimura 1998, CitationKlein et al. 1993, CitationDe Lange and Donath 1989). In the present study, unrestricted somatic stem cells (USSCs) were utilized as a regenerative cell for the growth and repairing of bone defect. Kogler and his colleagues isolated USSCs from umbilical cord blood, and evaluated the differentiation capacity and cytokine production of these cells for transplantation. In fact, USSCs are one of the rare cell populations in umbilical cord blood which are considered as pluripotent. Moreover, USSCs are highly potential for proliferation and differentiation. Therefore, USSCs are valuable sources for cell therapy (CitationMajdi et al. 2011, CitationRezaei-Tavirani et al. 2011, CitationAi et al. 2011, CitationHeidari et al. 2013, CitationZeinali et al. 2013, CitationBiazar and Heidari 2013). The purpose of this study was to investigate the bone-healing effects of injectable gel containing CS and HApnanoparticles combined with USSCs in calvarial bone of rats.
Materials and methods
Fabrication of injectable scaffold
The HAp: Ca10(PO4)6(OH)2) nanopowders (< 200 nm) were purchased from Sigma-aldrich Co. (USA). The CS gel was obtained by dissolving 1 g of CS in 50 ml of HCl solution (0.1 M), while the solution was being stirred using a magnetic stirrer till transparent. The nanoparticle powder sterilized by autoclave was added (0.01 g/mL) directly to the above stirring solution. Glycerophosphate powder, dissolved in distilled water (30% (w/v), was carefully added dropwise (with ultrasound (60 W)) to the resulting solution to obtain a clear and homogeneous liquid solution of nanoparticles/CS without precipitate (pH:7) .
Alkaline phosphatase (ALP) activity
The samples were washed with phosphate-buffered saline solution and transferred to 1.5 ml microtubes containing 1 ml ultra-pure water. Cell constructs were cryopreserved at − 80°C for further analysis. Prior to ALP quantification, the samples were thawed and sonicated for 15 min. ALP activity was measured by the specific conversion of p-nitrophenol phosphate (pNpp;Sigma) into p-nitrophenol (pNp). The enzymatic reaction was set up by mixing 100 ml of the sample with 300 ml of substrate buffer containing 1 M diethanolamine HCl, pH 9.8, and 2 mg/ml pNp. The solution was further incubated at 37°C for 1 h and the reaction stopped by adding a solution containing 2 M NaOH and 0.2 mM EDTA. The OD was determined at 405 nm.
Surgical procedures
From 30 male white wistar rats aged approximately 4–8 weeks at the beginning of the experiment and weighing 180–220 g, 30 rats were divided into three groups, each containing ten animals. The protocol for the experiment was approved by the Institutional Animal Care and Use Committee of Beheshti University (Iran). Animals were handled according to the guidelines established for animal care at the center. The calvarial defect model is convenient for examining bone regeneration because of its effective accessibility and lack of fixation requirements. Critical size defect in experimental models are essential bone reconstruction in in-vivo experiments (CitationZong et al. 2010, CitationHollingor and Kleinschmiolt 1990) also, the research and development on critical size defect attracted more attention in recent years (CitationKnabe et al. 2004). A cavity of about 5 mm wide, 5 mm long, and a depth of 8 mm was created by angel motor and terfain milling in calvarial bone of rats.
After preparation of all groups; the cells were harvested from tissue pleat culture, centrifuged (200 g) and mixed by rotator shaker. At last, this functional combination was pumped in to the bone defect. Finally 2 × 106 USSC per defect were used. The defects were filled with the designated scaffolds loaded with or without stem cells and skin sutured by using 5–0 nylon at 0.75 cm intervals. Among 30 of the bone defects, ten defects were filled with scaffold that was previously loaded with USSC at about 2 × 106 cells (Group A), scaffold or injectable gel without cell (Group B), control group (Group C) and then each animal was housed in its own cage to avoid damage to the wound. Post-surgery care included analgesic and antibiotic (cyclosporine) injections. Checking of any post-surgery pain, distress or complications was done 24 h after surgery and daily afterwards.
Computed tomography (CT) analysis
A CT scanner (3 Tesla, Siemens Sensation 16 Slice CT) was used to determine bone growth occurring in the engineered constructs. Specimens were scanned with a spatial resolution: 30 lp/cm and reconstruction time per image: 0.1 s. Samples were also analyzed using a syngo software and therefore areas of bone density were identified.
Histological assessment
For observation of the tissue structures and thickness of the new bone, histological examinations were carried out. Samples were fixed with formalin 10% for 2 weeks. Subsequently, the samples were processed for histological examination according to standard procedures. Briefly, the samples were embedded in paraffin, sectioned at 4-μm thickness and subjected to hematoxylin–eosin (HE) staining. All the stained sections were observed by optical microscope (Nikon; Japan). After 4 weeks, the reconstructed area was analyzed by histomorphometric measurements of the width of the repaired area and then the correlation coefficient (r = 1,0;p < 0.05) and detected rebuilding rate were calculated. The reconstructed area less than 20% (faulty healing), reconstructed area between 20% and 60% (partially healing) and more than 60% (significant healing) were defined in this analysis. Experimental results were expressed as means ± SD. All data were analyzed by one-way analysis of variance (ANOVA) with Duncan's multiple range tests (ANOVA; Duncan multiple range test, p < 0.05 and 0.01).
Results and discussion
ALP activity
The ALP activity of USSCs cultured onto the scaffolds did not follow the typical trend of this marker of osteogenic differentiation, as it was demonstrated after 28 days. After this time period, a significant increase in ALP activity was observed (). Usually, ALP reaches a peak at an earlier time period.
Results of CT-Scan
After 4 weeks, before CT-scan, the rats were deeply anesthetized with xylene and ketamine then, they were placed inside the inhibitor box and the rebuild area was evaluated by CT-SCAN (3Tesla). The figures show reconstructing coordination with the scaffolds. The CT results suggested that after 4 weeks of implantation, cell constructs promoted bone regeneration of the calvarial defect (). The CT images also supported the enhanced bone ingrowth in scaffolds cultured with USSCs. Some of the images clearly show an almost complete healing of the defect (). The stem cells loaded in scaffolds were able to induce some bone regeneration/ingrowth. New bone formation could be due to invading reparative cells from the dura or from adjacent host tissues. The selected implantation time seemed to be adequate for assessing the complete bone healing at the defected site, as shown in . shows the regeneration of calvarial bone in rats that was obtained by CT analysis.
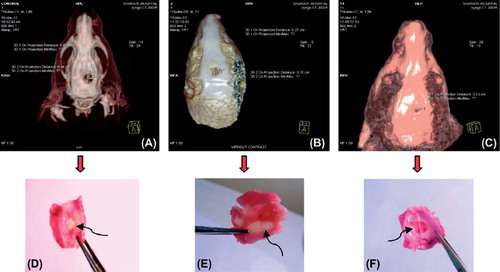
Table I. Results of bone regeneration in calvarial bone obtained by CT analysis at 4th week.
Histological results
Typical HE-stained histological sections at 4 weeks after implantation are shown in . After 4 weeks, in control sample, fibrous tissue extends from the defect margin with a few small areas of new bone formation (≤ 10%) visible immediately adjacent to the host bone, indicating unsuccessful efforts at regeneration, while histomorphometric results showed that more than 50% reconstruction has occurred in calvaria for the scaffold with cells after 28 days. The new bone formation was both continuous and discontinuous with the calvarial bone. The new bone was thin and the tissue structure was incomplete for the scaffold. Bone formation increased for the scaffold/USSC group. The thickness and structure of the new bone were better in the scaffold/USSC group than the scaffold group. In the scaffold group without cells, the continuity of the bone regeneration was incomplete. Ossification rate for the samples with USSC is more than the other groups also, bone quality and relevance of new bone tissue and surrounding bone tissue were established for the scaffold/USSC group. None of the samples showed any marked inflammatory reactions caused by the bonding agent or other materials.
Conclusion
In the present study, the bone-healing effects of injectable CS/HAp nanoparticles gel with USSCs grafted in rat calvarial model were investigated. In addition, bone regeneration was achieved using the injectable scaffold especially with stem cells in rat calvarial bone. This study evidenced very positive results that highlight the possibility of injectable scaffolds especially import effect of USSCs to be used as effective design for healing non-load-bearing bone defects.
Declaration of interest
The authors report no declarations of interest. The authors alone are responsible for the content and writing of the paper.
References
- Ai J, Heidari SK, Ghorbani F, Ejazi F, Biazar E, Asefnejad A, Pourshamsian K, et al. 2011. Fabrication of coated-collagen electrospun PHBV nanofiber film by plasma method and its cellular study. J Nanomater 2011:1–8.
- Biazar E, Heidari SK. 2013. Effects of chitosan cross linked nanofibrous PHBV scaffold combined with mesenchymal stem cells on healing of full-thickness skin defects. J Biomed Nanotechol. 9:1471–1482.
- Branski LK, Gauglitz GG, Herndon DN, Jeschke MG. 2009. A review of gene and stem cell therapy in cutaneous wound healing. Burns.35:171–180.
- Chesnutt BM, Viano AM, Yuan Y, Yang Y, Guda T, Appleford MR, et al. 2009a. Design and characterization of a novel chitosan/nanocrystalline calciumphosphate composite scaffold for bone regeneration. J Biomed Mater Res A. 88:491–502.
- Chesnutt BM, Yuan Y, Buddington K, Haggard WO, Bumgardner JD. 2009b. Composite chitosan/nano-hydroxyapatite scaffolds induce osteocalcin production by osteoblasts in vitro and support bone formation in vivo. Tissue Eng Part A.15:2571–2579.
- De Lange GL, Donath K. 1989. Interface between bone tissue and implants of solid hydroxyapatite or hydroxyapatite-coated titanium implants. Biomaterials. 10:121–125.
- Di Martino A, Sittinger M, Risbud MV. 2005. Chitosan: A versatile biopolymer for orthopaedic tissue-engineering. Biomaterials. 26: 5983–5990.
- Heidari SK, Biazar E, Rezaei-Tavirani M, Rahmati-Roodsari M, Ronaghi A, Ebrahimi M, et al. 2013. The healing effect of unrestricted somatic stem cells loaded in collagen-modified nanofibrous PHBV scaffold on full-thickness skin defects. Artif Cell Nanomed Biotech. (Epub ahead of print).
- Hollingor JO, Kleinschmiolt JC. 1990. The critical size defect as an experimental model to test bone repaiur materials. J Craniofac Surg.1:60–68.
- Horwitz EM, Prockop DJ, Fitzpatrick LA, Koo WW, Gordon PL, Neel M, et al. 1999. Transplantability and therapeutic effects of bone marrow-derived mesenchymal cells in children with osteogenesis imperfect. Nat Med.5:309–313.
- Huang Z, Tian J, Yu B, Xu Y, Feng Q. 2009. A bone-like nano- hydroxyapatite/collagen loaded injectable scaffold. Biomed Mater. 4:550–555.
- Ishige I, Nagamura-Inoue T, Honda MJ, Harnprasopwat R, Kido M, Sugimoto M, et al. 2009. Comparison of mesenchymal stem cells derived from arterial, venous,and Wharton's jelly explants of human umbilical cord. Int J Hematol. 90:261–269.
- Jayakumar R, Prabaharan M, Nair SV, Tamura H. 2010. Novel chitin and chitosan nanofibers in biomedical applications. Biotechnol Adv. 28:142–150.
- Kamolz LP, Kolbus A, Wick N, Mazal PR, Eisenbock B, Burjak S, Meissl G. 2006. Cultured human epithelium: human umbilical cord blood stem cells differentiate into keratinocytes under invitro conditions. Burns. 32:16–19.
- Khor E, Lim LY. 2003. Implantable applications of chitin and chitosan. Biomaterials. 24:2339–2349.
- Kim IY, Seo SJ, Moon HS, Yoo MK, Park IY, Kim BC, Cho CS. 2008. Chitosan and its derivatives for tissue engineering applications. Biotechnol Adv. 26:1–21.
- Kita K, Gauglitz GG, Phan TT, Herndon DN, Jeschke MG. 2010. Isolation and characterization of mesenchymal stem cells from the sub-amniotic human umbilical cord lining membrane. Stem Cells Dev. 19:491–502.
- Klein C, Wolke JGC, Groot D. 1993. Stability of Calcium Phosphate Ceramics and Plasma Sprayed Coating. In: Hench LL, Wilson J, Eds. An Introduction to Bioceramics. Singapore: World Scientific Publishing.
- Knabe C, Berger G, Gildenhaar R, Meyer J, Howlett CR, Markovic B, Zreiqat H. 2004. Effect of rapidly resorbable calcium phosphates and a calcium phosphate bone cement on the expression of bone-related genes and proteins in vitro. J Biomed Mater Res A. 69: 145–154.
- Liu D, Troczynski T, Wenjea T. 2001. Water-based sol-gel synthesis of hydroxyapatite: process development. Biomaterials. 22:1721–1730.
- Majdi A, Biazar E, Heidari S. 2011. Fabrication and comparison of electro-spun PHBV nanofiber and normal film and its cellular study. Orient. J Chem. 27:523–528.
- Meirelles LS, Chagastelles PC, Nardi NB. 2006. Mesenchymal stem cells reside in virtually all post-natal organs and tissues. J Cell Sci. 119:2204–2213.
- Monteiro BS, Argolo-neto NM, Nardi NB. 2012. Treatment of critical defects produced in calvaria of mice with mesenchymal stem cells. An Acad Bras Cienc. 84:841–851.
- Reves BT, Bumgardner JD, Cole JA, Yang Y, Haggard WO. 2009. Lyophilization to improve drug delivery for chitosan-calcium phosphate bone scaffold construct: A preliminary investigation. J Biomed Mater Res B. 90:1–10.
- Rezaei-Tavirani M, Biazar E, Ai J, Heidari S, Asefnejad A. 2011. Fabrication of collagen-coated poly (beta-hydroxy butyrate-co- beta-hydroxyvalerate) nanofiber by chemical and physical methods. Orient J Chem. 27:385–395.
- Shi C, Zhu Y, Ran X, Wang M, Su Y, Cheng T. 2006. Therapeutic potential of chitosan and its derivatives in regenerative medicine. J. Surg Res. 133:185–192.
- Suchanek W, Yoshimura M. 1998. Processing and properties of hydroxyapatite-based biomaterials for use as hard tissue replacement implants. J Mater Res. 3:94–117.
- Venkatesan J, Kim SK. 2010. Chitosan composites for bone tissue engineering—An overview. Mar Drugs8:2252–2266.
- Wang F, Li M, Lu Y, Qi Y. 2005. A simple sol–gel technique for preparing hydroxyapatite nanopowders. Mater Lett. 59:916–919.
- Wang M. 2003. Developing bioactive composite materials for tissue replacement. Biomaterials. 24:2133–2151.
- Wilson A, Butler PE, Seifalian AM. 2011. Adipose-derived stem cells for clinical applications. Cell Prolif. 44:86–98.
- Wu KH, Zhou B, Lu SH, Feng B, Yang SG, Du WT, et al. 2007. In vitro and in vivo differentiation of human umbilical cord derived stem cells into endothelial cells. J Cell Biochem. 100:608–616.
- Zeinali R, Biazar E, Heidari S, Tavirani M, Asadipour K. 2013. Regeneration of Full-Thickness Skin Defects Using Umbilical Cord Stem Cells Loaded into Modified Porous Scaffolds. ASAIO. 60:106–114.
- Zhang Y, Zhang M. 2001. Synthesis and characterization of macroporous chitosan/calcium phosphate composite scaffolds for tissue engineering. J Biomed Mater Res. 55:304–312.
- Zong C, Xue D, Yuang W, Wang W, Shen D, Tong X, et al. 2010. Reconstruction of rat calvarial defects with human mesenchymal stem cells and osteoblast – like cells in poly – lactic- co- Glycolic Acid scaffolds. J Erop Cell Mater. 20:109–120.