Abstract
Context: PEGylated hemoglobin (Hb) is a promising oxygen therapeutic agent for clinical application. However, it suffered from structural perturbation, functional instability and methemoglobin (metHb) formation. Objective: To improve the structural, functional, physical and anti-oxidation properties of the PEGylated Hb. Materials and methods: PEGylation of Hb with CO binding (HbCO) was conducted using maleimide and acylation chemistry, respectively. Physical and chemical parameters were measured for Hb samples. The circular dichroism spectra, dynamic light scattering and analytical ultracentrifugation were used to investigate the structure and conformation of PEGylated HbCO. Results: CO binding can inhibit the autoxidation of the PEGylated Hb, structurally stabilize its tetramer and improve its thermal and pH stability. Importantly, the circular dichroism spectra showed that CO binding can decrease the structural perturbation of Hb induced by PEGylation. The PEGylated HbCO with CO release showed slightly higher oxygen-delivery capacity than the PEGylated Hb. The PEGylated HbCO did not show metHb formation after 30-day storage at 4°C. Discussion and conclusion: CO binding structurally stabilized the PEGylated Hb, abolished its metHb formation, and significantly increased its physical stability. In particular, it also avoided the perturbation of PEG chains on the heme microenvironment. The functional property of the PEGylated HbCO can be maintained during its long-term storage, which is of great significance for field transfusion.
Introduction
PEGylation, chemical conjugation with polyethylene glycol (PEG), can reduce renal clearance, enzymatic degradation and immunogenicity of peptides and proteins, thereby improving their therapeutic potentials (CitationPasut and Veronese 2012, CitationXue et al. 2013, CitationMu et al. 2013). Hemoglobin (Hb) is a potential therapeutic agent as oxygen carrier and a protein responsible for storage and transport of oxygen in red blood cell (CitationRameez et al. 2008, CitationTsuchida et al. 2009). However, Hb suffers from the disadvantages of nephrotoxicity, short in vivo half-life and vasoactivity (CitationCole and Vandegriff 2011, CitationElmer et al. 2012). Animal tests and clinical trials suggest that PEGylation has emerged as a novel chemical approach to circumvent these disadvantages of Hb (CitationJahr et al. 2012, CitationHu et al. 2007). Therefore, the PEGylated Hb is a potent candidate for development of Hb based oxygen carriers (HBOC).
Hb in a ferrous state can be easily oxidized to methemoglobin (metHb) in a ferric state. MetHb may lose its oxygen-delivery capacity, release its cytotoxic heme in the circulation and generate harmful reactive oxygen species (ROS) (CitationAlayash et al. 2001, CitationVandegriff et al. 2006, CitationDunne et al. 2006). Hb also undergoes in vivo rapid dissociation from its native tetrameric state (α2β2 tetramer) to dimeric state (αβ dimer), which leads to nephrotoxicity (CitationLunelli et al. 1994). Recent studies suggested that PEGylation can accelerate the autoxidation of Hb (CitationHu et al. 2008, Citation2011). On one hand, the heme environment of Hb may be structurally perturbed by PEGylation, particularly at the sites proximal to the heme pocket, such as at Cys-93(β) (CitationHuang et al. 2012). On the other hand, the conjugated PEG chains can act as a reservoir of waters in the hydration layer of Hb (CitationHu et al. 2005) and enhance the oxidation of Hb by promoting nucleophilic attack of heme (CitationHu et al. 2008). Typically, PEGylation of Hb was conducted in an air-equilibrated environment and metHb formation may occur in the PEGylation process. PEGylation in anaerobic condition has been carried out in the presence of Cl− and inositol hexaphosphate (IHP) to inhibit the metHb formation of Hb (CitationIafelice et al. 2007). However, achieving such an oxygen-free environment requires considerable effort to remove O2 from Hb. PEGylation also induces extensive tetramer-dimer dissociation of Hb, thereby resulting in significant structural and functional perturbation of Hb (CitationHu et al. 2007, Citation2009). For example, tetramer-dimer dissociation of Hb unfavorably alters the heme environment and quaternary structure and destabilizes the α1β2 interface of Hb (CitationCaccia et al. 2009).
It is well known that the bond between heme of Hb and CO is much more stable than that between heme and O2 (CitationBalakrishnan et al. 2009). This implies that CO binding with heme can inhibit the autoxidation of Hb and improve the physical stability of Hb. For example, MP4 (Sangart Inc., USA), a PEGylated Hb with ˜ 7 copies of 5 kDa PEG conjugated to human Hb, has displayed an increased stability during storage upon CO binding (CitationVandegriff et al. 2008). Since the PEGylated Hb with CO binding (HbCO) cannot be oxidized, its autoxidation may be avoided and the long-term storage of the PEGylated Hb can be achieved by CO binding. Thus, CO binding is a possible approach to decrease or circumvent the above side effects of the PEGylated Hb.
In the present study, we have investigated the effect of CO binding on the structural (heme environment and molecular shape, etc.), functional (oxygen affinity and Hill coefficient), physical (pH and thermal stability), and anti-oxidation properties of the PEGylated Hb. PEGylation of HbCO was conducted using acylation chemistry and maleimide chemistry, respectively. Acylation chemistry can stochastically modify the ε-NH2 of Hb and has been used to prepare the Enzon's product (Enzon Inc., USA) (CitationConover et al. 1996). Maleimide chemistry can specifically modify Cys-93(β) of Hb and has been used to prepare MP4 (Sangart Inc., USA) (CitationWinslow 2004). Our study is expected to decrease or circumvent the side effect of the PEGylated Hb by CO binding.
Materials and methods
Materials
Carbon monoxide (99.9%) and oxygen (99.9%) were purchased from Yanan Gas Technology Co., Ltd. (Beijing, China). PEG succinimidyl carbonate (PEG-sc, 5 kDa) and PEG maleimide (PEG-mal, 5 kDa) were provided by the National Research Center for Biotechnology (Beijing, China).
PEGylation of Hb and HbCO
Human Hb was purified from erythrocytes as described previously (CitationLu et al. 2004). The purified Hb in PBS buffer (pH 7.4) was bubbled with CO to obtain HbCO. HbCO (0.15 mM in PBS buffer, pH 7.4) and Hb (0.15 mM in PBS buffer, pH 7.4) were allowed to react with 20-fold molar excess of PEG-sc polymers with PEG mass of 5 kDa at 4°C overnight, respectively. The ε-NH2 groups of Hb were stochastically modified by PEG-sc. Hb (0.15 mM in PBS buffer, pH 7.4) and HbCO (0.15 mM in PBS buffer, pH 7.4) were also allowed to react with a 10-fold molar excess of PEG5k-mal respectively, and the reaction mixtures were incubated at 4°C overnight. The thiol groups of Cys-93(β) of Hb were specifically modified by PEG-mal. The reaction mixture was loaded on a DEAE Sepharose Fast Flow column (1.6 × 10 cm, GE Healthcare, USA). The column was equilibrated and eluted with 50 mM Tris-Ac buffer (pH 8.5) to remove the free PEG reagent. The bound PEGylated Hb was eluted from the column with 50 mM Tris-Ac buffer (pH 7.0).
Analytical methods
Size exclusion chromatography (SEC) analysis was carried out on a Superdex 200 column (1 × 30 cm, GE Healthcare, USA). The column was equilibrated and eluted with PBS buffer (pH 7.4) at a flow rate of 0.5 ml/min. SDS-PAGE analysis was carried out on a 15% polyacrylamide gel and the gel was stained with Coomassie Blue R-250. Spectrometric analysis was performed on a double-beam spectrophotometer (Model U-3110, Hitachi, Japan).
Circular dichroism spectroscopy
Circular dichroism (CD) spectra were recorded on a J-810 spectropolarimeter (Jasco, Japan) using a 1-mm path length cuvette at 25°C. The spectra of buffer blanks were measured before the samples and subtracted from the spectra of the samples. The CD spectra (250–480 nm) were obtained using 26.0 μM Hb samples (in tetramer). Far UV CD spectra (200–250 nm) were obtained using 1.3 μM Hb samples (in tetramer). The molar ellipticity (θ) was expressed in deg cm2/dmol on a heme basis.
Autoxidation
Autoxidation experiments were carried out by incubation of the Hb samples (25 μM as tetramer) in PBS buffer (pH 7.4) containing 300 μM EDTA at 37°C. Aliquots of the samples were taken out at various time intervals. Total Hb concentration was calculated using ε523 = 7.12 mM−1 cm−1 (CitationSnell and Marini 1988). The methemoglobin (metHb) level was measured based on absorbance at 560, 576, 630 and 700 nm (CitationBenesch et al. 1973). Spectrophotometric measurements were carried out in a double-beam spectrophotometer (Model U-3110, Hitachi, Japan). The first-order autoxidation rate constants (kox) were measured as CitationHu et al. (2008).
Oxygen affinity and Hill coefficient
The HbCO samples were converted to oxygenated state by exposure to an O2 atmosphere under irradiation of a visible light (CitationSakai et al. 1993a). In addition, the Hb and HbCO samples were stored at 4°C for 30 days. The resultant HbCO samples were converted to oxygenated state. Oxygen equilibrium curves of the Hb and HbCO samples were carried out using a Hemox Analyzer (TCS Corp., PA, USA) in Hemox buffer (pH 7.4) at 37°C. The O2 equilibrium curves for each sample were used to obtain the values of Hill coefficient (n) and the oxygen pressure at which Hb was half saturated (P50).
The pH stability
The pH stability of the Hb and HbCO samples was investigated by measuring the far UV CD spectra of the samples under different pH conditions. The ellipticity at 222 nm of each sample was recorded, as the ellipticity at 222 nm can reflect the α-helix content of Hb (CitationKelly and Price 2000). Here, the α-helix content of Hb at pH 7.4 was set as 100%. The samples were at Hb concentration of 1.3 μM (in tetramer).
Thermal stability
The Hb and HbCO samples were heated at 65°C as a function of incubation time (CitationRuckpaul et al. 1971). Briefly, each Hb sample (9.0 ml, 2 mg/ml) in PBS buffer (pH 7.4) was divided into 9 aliquots of 1 ml. Each aliquot was kept in tightly-capped microcentrifuge tubes and heated at 65°C in a water bath. The tubes were removed from the water bath at 0, 5, 10, 15, 20, 30, 40, 50 and 60 min, respectively. The tubes were immediately incubated in an ice bath for 10 min and centrifuged at 10,000 g for 10 min, followed by removal of the precipitate. The absorbance at 523 nm of the supernatant was used to calculate the percent denaturation from the equation: Denaturation (%) = (A0−At)/A0 × 100, where A0 is the absorbance of the unheated aliquot at 523 nm and At is the absorbance of the heated sample at 523 nm at time t.
The Hb and HbCO samples were heated for 30 min as a function of temperature and then immediately incubated in an ice bath for 10 min. The resultant samples were centrifuged at 10,000 g for 10 min, followed by removal of the precipitate. The supernatant was subjected to CD analysis in the far UV region (200–250 nm). The ellipticity at 222 nm of each sample was recorded. The retention of secondary structure was calculated from the equation: Retention (%) = ([θ]−[θ]0)/[θ]0 × 100%, where [θ]0 and [θ] are the ellipticity values of the unheated and heated samples at 222 nm, respectively.
Dynamic light scattering
Dynamic light scattering analysis was performed using a Wyatt DynaPro Titan (Santa Barbara, CA) at 25°C. The samples were at a protein concentration of 1.0 mg/ml and centrifuged at 15,700 g for 10 min before analysis.
Sedimentation velocity
Sedimentation velocity was detected by analytical ultracentrifugation on a ProteomeLab XL-1 (Beckman, USA) equipped with an An-60Ti rotor. The samples at the nominal concentration (A280 = 0.740) were centrifuged at 60,000 g in PBS buffer (pH 7.4) at 20°C. Boundary movement was detected at 280 nm using the absorption optics. The sedimentation coefficient (S) and the ratio of frictional coefficient (f/f0) were calculated using v– of 0.744 ml/g for Hb and 0.806 ml/g for the PEGylated proteins (CitationKellett 1971). These values were normalized to standard conditions.
Results
Characterization of the PEGylated proteins
The PEGylated proteins were characterized by size exclusion chromatography (SEC). As shown in , Hb was eluted as a single and symmetric peak. The elution peak of Hb was left-shifted upon PEGylation. This indicated that PEGylation can increase the hydrodynamic volume of Hb. Moreover, the PEGylated Hb prepared with PEG-sc (P-sc-Hb) was eluted earlier than that with PEG-mal (P-mal-Hb), due to the more conjugated PEG chains in P-sc-Hb. However, the elution peaks of Hb, P-sc-Hb and P-mal-Hb were not shifted upon CO binding. This indicated that CO binding did not alter the hydrodynamic volumes of the Hb samples.
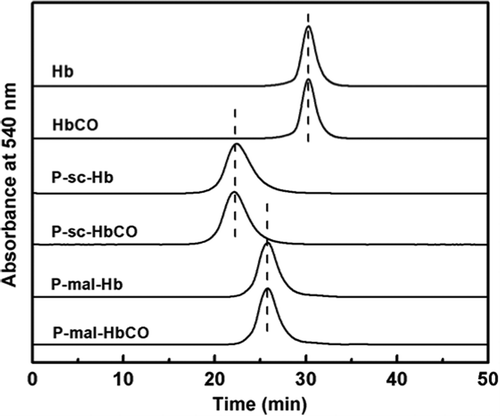
The molecular radii of the Hb and HbCO samples were determined by dynamic light scattering. As shown in , PEGylation can significantly increase the molecular radii and volumes of Hb and HbCO. However, the molecular volume of HbCO is comparable to that of Hb. Similarly, the molecular volumes of P-sc-HbCO and P-mal-HbCO were comparable to those of P-sc-Hb and P-mal-Hb, respectively. This was consistent with the result of SEC analysis.
Table I. The radius and molecular volume of the PEGylated proteins.a
As shown in , P-mal-Hb (Lane 4) showed an additional band with respect to Hb (Lane 2). This was due to that Cys-93(β) in β globin of Hb was specifically conjugated with PEG-mal, and α globin of Hb was unmodified. P-sc-Hb (Lane 3) showed additionally one major band and one minor band with respect to Hb. This indicated that Hb was non-specifically conjugated with PEG-sc, and partial globins were unmodified. P-sc-HbCO (Lane 6) exhibited migration bands comparable to P-sc-Hb (Lane 3). Similarly, the migration bands of P-mal-HbCO (Lane 7) and HbCO (Lane 5) were comparable to those of P-mal-Hb (Lane 4) and Hb, respectively. This indicated that CO binding did not alter the PEGylation pattern of Hb.
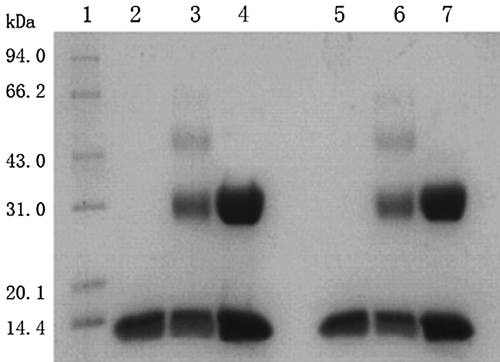
As shown by spectrometric analysis (), Hb showed three peaks at 414, 540 and 575 nm. The three peaks of Hb were shifted to 419, 538 and 569 nm upon CO binding. The optical shift of Hb indicated the successful binding of CO with the heme of Hb. In contrast, the optical spectra of Hb and HbCO were not further altered upon PEGylation. This indicated that PEGylation did not alter the optical absorption of Hb and HbCO.
Structural analysis
Circular dichroism spectroscopy
In the near UV and Soret band region (250–500 nm), CO binding and PEGylation both altered the CD spectra of Hb (). L band (around 260 nm) is considered to be sensitive to the interactions between heme and the surrounding globin. PEG-sc mediated PEGylation apparently increased the L band of Hb (). This suggested that PEGylation unfavorably altered the heme environment of Hb. In contrast, the L band of P-sc-Hb was decreased upon CO binding (). This suggested that CO binding can decrease the structural perturbation of the heme environment induced by PEGylation.
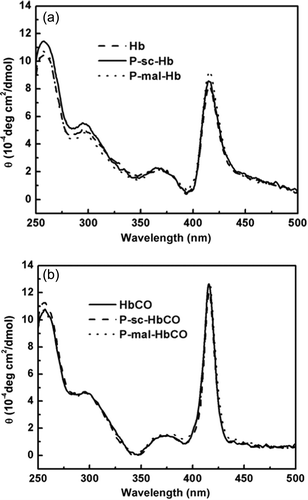
The Soret band (around 420 nm) of Hb is informative regarding the interactions of the heme prosthetic group with the surrounding aromatic residues. The modifications of these residues in the spatial orientation around the heme may affect porphyrin transitions and π-π* transitions in the surrounding aromatic residues. As reflected by the apparent increase in the Soret bands of P-mal-Hb and P-sc-Hb, the conjugated PEG chains unfavorably altered the interactions between the heme and the surrounding globin chain of Hb (). In contrast, the Soret bands of P-mal-Hb and P-sc-Hb were essentially superimposed on that of HbCO (). This CO binding can decrease the structural perturbation of Hb induced by PEGylation.
Sedimentation velocity
Sedimentation velocity studies were conducted to gain the conformational information of the Hb and HbCO samples. Sedimentation coefficients (S20,w) can be used to monitor the conformational change of proteins (CitationCarvalho et al. 2011). As shown in , the S20,w of Hb decreased upon PEGylation, due to the hydrodynamic drag of the PEG chains (i.e., the parachute effect of PEG). P-sc-HbCO and P-mal-HbCO showed higher S20,w values than P-sc-Hb and P-mal-Hb, respectively. This indicated that CO binding structurally altered P-sc-Hb and P-mal-Hb, thereby increasing their molecular density. In addition, P-sc-HbCO showed a lower S20,W than P-mal-HbCO, due to more conjugated PEG chains in P-sc-Hb.
Table II. The sedimentation velocity and f/f0 of the PEGylated proteins.
The ratio of frictional coefficient (f/f0) can be used to provide hydrodynamic shape information of macromolecules (CitationLi et al. 2012). As shown in , PEGylation can increase the f/f0 of Hb, indicating the non-ideality and molecular asymmetry of P-sc-Hb and P-mal-Hb. P-sc-HbCO and P-mal-HbCO showed lower f/f0 values than P-sc-Hb and P-mal-Hb, respectively. This indicated that CO binding can decrease the f/f0 of P-sc-Hb and P-mal-Hb. Presumably, CO binding can tighten the tetrameric structure of P-sc-Hb and P-mal-Hb, thereby increasing their molecular symmetry and structurally stabilizing Hb.
Physical stability of the PEGylated proteins
The pH stability
The pH stability of the Hb and HbCO samples was evaluated by measuring their α-helix contents under different pH conditions. The α-helix content of Hb was stable at pH 5.0–8.5 (data not shown). All the Hb samples showed a decrease in the α-helix content at pH 3.0 (), because Hb samples were unstable under acid condition. However, PEGylation and CO binding both can increase the retention in the α-helix contents of Hb at pH 3.0. Moreover, CO binding had higher ability than PEGylation to enhance the pH stability of Hb.

Thermal stability
Thermal stability of the Hb and HbCO samples was investigated as a function of incubation time at 65°C. As shown in , PEGylation can decrease the denaturation rate of Hb. Moreover, CO binding can significantly decrease the denaturation rate of P-sc-Hb and P-mal-Hb. In contrast, the denaturation rate of P-mal-Hb was higher than that of HbCO. Thus, the ability of CO binding to increase the thermal stability was higher than that of PEGylation.
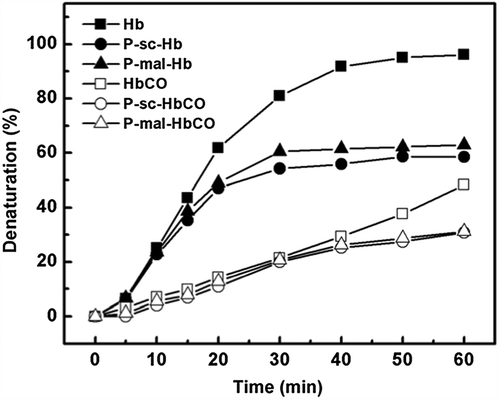
Thermal stability of the Hb and HbCO samples was also investigated as a function of incubation temperature for 30 min. As shown in , PEGylation and CO binding both can increase the retention in the α-helix of Hb upon heat treatment. As shown in , the Tm value of Hb can be increased by PEGylation and by CO binding. P-sc-HbCO showed the highest Tm in the samples, reflecting the synergistic effect of PEGylation and CO binding on the thermal stability of Hb. Since the Tm difference between P-sc-HbCO and P-sc-Hb is much higher than the one between P-sc-Hb and Hb, CO binding had higher ability than PEGylation to increase the thermal stability of Hb.
Anti-oxidation property
The metHb formation kinetics of the Hb and HbCO samples was shown in . P-sc-Hb showed a metHb content higher than Hb and lower than P-mal-Hb during the incubation period at 37°C. Hb displayed a first-order autoxidation rate constant (kox) of 0.012 h−1. In contrast, P-mal-Hb and P-sc-Hb displayed the kox of 0.020 h−1 and 0.016 h−1, respectively. This indicated that PEGylation can enhance the autoxidation of Hb. Moreover, PEGylation at Cys-93(β) that was proximal to the heme pocket can significantly increase the autoxidation of Hb. In contrast, CO binding of the Hb samples can abolish the occurrence of autoxidation, as reflected by the fact that metHb was essentially not observed in the HbCO samples. Presumably, CO binding can prevent ferrous (Fe2+) heme from the nucleophilic attack, thereby essentially inhibiting the metHb formation.
Functional property
The functional property of the HbCO samples with CO release was measured and compared with that of the Hb samples. As shown in , Hb showed a P50 (oxygen affinity) of 13.0 mmHg with Hill coefficient (n) of 2.8. P-sc-Hb showed a P50 of 7.0 mmHg with n of 2.0. P-mal-Hb showed a P50 of 8.2 mmHg with n of 2.1. This suggested that PEGylation can increase the oxygen affinity of Hb. Interestingly, the HbCO samples with CO release showed slightly higher P50 and n than the corresponding Hb samples. Presumably, CO binding can structurally stabilize Hb and improve the anti-oxidation property of Hb, which in turn improved the functional property of the Hb samples. The metHb contents in the Hb samples were over 15% after storage at 4°C for 30 days, which led to complete loss of oxygen-delivery capacity of the Hb samples. In contrast, the HbCO samples under this storage condition can maintain the oxygen-delivery capacity after CO release.
Table III. Oxygen-delivery properties of the PEGylated proteins.
Discussion
Our present study was aimed to decrease or circumvent some side effects of the PEGylated Hb, such as structural perturbation, functional instability and autoxidation. Accordingly, CO binding was used to improve the structural, physical, functional and anti-oxidation properties of the PEGylated Hb.
Structurally, CO binding can alter the heme environment and molecular shape of the PEGylated Hb. Heme environment, the nonpolar environment around heme, can prevent heme from the contact with water or other polar molecules, thereby inhibiting oxidation of Fe2+ to Fe3+. Previous report suggested that the heme environment of Hb was perturbed by conjugation of the PEG chains, especially conjugation at the sites proximal to the heme pocket, such as at Cys-93(β) (CitationLi et al. 2009). Our present study suggested that the heme environment of Hb can be structurally stabilized upon CO binding. Presumably, the relative position of porphyrin with the surrounding aromatic residues is changed upon CO binding and the side chain of Cys-93(β) points away from the surface of Hb, thereby stabilizing the Hb structure (CitationLi et al. 2009). Our study also suggested that CO binding can slightly tighten the tetrameric structure of the PEGylated Hb and structurally stabilize the Hb tetramer, as reflected by its symmetric molecular shape and increased molecular density. Presumably, CO binding may interrupt the conformational transition of Hb from R state to T state.
Physically, CO binding can improve the thermal stability and pH stability of the PEGylated Hb in the present study. Consistently, CitationSakai et al. (1993b) reported that endothermic transition points of oxyHb and HbCO were observed at 62.2°C and at 77.7°C, respectively. Presumably, the energy barrier to break the heme-protein bond is greater in the PEGylated HbCO than that in the PEGylated Hb. Thus, higher temperature is needed to destabilize the PEGylated HbCO, as compared with the PEGylated Hb. In addition, the PEGylated HbCO is slightly more resistant to low pH than the PEGylated Hb. The higher stability of PEGylated HbCO may come from the tighter conformation of the HbCO protein core, which makes all the salt bonds and hydrogen bonds inside HbCO molecule more stable.
MetHb formation gives rise to a decrease in the oxygen-delivery capacity, generation of reactive oxygen species (ROS) and heme loss of Hb (CitationVandegriff et al. 2006) . Moreover, the PEG chains can increase the number of water molecules in the hydration layer of Hb and enhance the autoxidation by promoting the nucleophilic attack of heme. PEGylation may also alter the heme environment of oxyHb and increase the solvent accessibility to the heme pocket, thereby promoting the metHb formation (CitationHu et al. 2008). Our present study suggested that the autoxidation of the PEGylated Hb can be abolished upon CO binding. CO binding can strongly stabilize the bond between heme and CO and keep the iron atoms in the low-spin ferrous state (CitationBaldwin 1980). This can prevent heme from the nucleophilic attack and avoid the autoxidation of the PEGylated Hb.
Functionally, the oxygen-delivery capacity of the PEGylated HbCO could be recovered after O2 feeding under visible light irradiation (CitationSakai et al. 2011, CitationWinslow 2000). Our present study suggested that the PEGylated HbCO with CO release even showed higher functional property than the PEGylated Hb. Interestingly, the PEGylated HbCO with CO release can maintain its oxygen-delivery capacity during long-term storage at 4°C. Moreover, metHb was not observed during this period. Thus, CO binding was useful for long-term storage of PEGylated Hb. Similarly, CO-MP4 did not oxidize and showed essentially no met-Hb formation over time (CitationVandegriff et al. 2008).
In summary, CO binding structurally stabilized the PEGylated Hb, abolished its metHb formation, and significantly increased its physical stability. In particular, the functional property of the PEGylated HbCO can be maintained during its long-term storage, which is of great significance for field transfusion.
Acknowledgments
The authors thank National Key Basic Research Program, No. 2013CB733604, Natural Science Foundation of China (Grant 21336010 and Grant 20906095) and 863 High-Tech Project, 2012AA02A406.
Declaration of interest
The authors report no declarations of interest. The authors alone are responsible for the content and writing of the paper.
References
- Alayash AI, Patel RP, Cashon RE. 2001. Redox reactions of hemoglobin and myoglobin: biological and toxicological implications. Antioxid Redox Signal. 3:313–327.
- Balakrishnan G, Zhao X, Podstawska E, Proniewicz LM, Kincaid JR, Spiro TG. 2009. Subunit-selective interrogation of CO recombination in carbonmonoxy hemoglobin by isotope-edited time-resolved resonance Raman spectroscopy. Biochemistry. 48:3120–3126.
- Baldwin JM. 1980. The structure of human carbonmonoxy haemoglobin at 2.7 A resolution. J Mol Biol. 136:103–128.
- Benesch RE, Benesch R, Yung S. 1973. Equations for the spectrophotometric analysis of hemoglobin mixtures. Anal Biochem. 55:245–248.
- Caccia D, Ronda L, Frassi R, Perrella M, Del Favero E, Bruno S, et al. 2009. PEGylation promotes hemoglobin tetramer dissociation. Bioconjugate Chem. 20:1356–1366.
- Carvalho FA, Santiago PS, Borges JC, Tabak M. 2011. Molecular masses and sedimentation coefficients of extracellular hemoglobin of Glossoscolex paulistus: alkaline oligomeric dissociation. Int J Biol Macromol. 48:183–193.
- Cole RH, Vandegriff KD. 2011. MP4, a vasodilatory PEGylated hemoglobin. Adv Exp Med Biol. 701:85–90.
- Conover CD, Malatesta P, Lejeune L, Chang CL, Shorr RG. 1996. The effects of hemodilution with polyethylene glycol bovine hemoglobin (PEG-Hb) in a conscious porcine model. J Investig Med. 44:238–246.
- Dunne J, Caron A, Menu P, Alayash AI, Buehler PW, Wilson MT, et al. 2006. Ascorbate removes key precursors to oxidative damage by cell-free haemoglobin in vitro and in vivo. Biochem J. 399:513–524.
- Elmer J, Alam HB, Wilcox SR. 2012. Hemoglobin-based oxygen carriers for hemorrhagic shock. Resuscitation. 83:285–292.
- Hu T, Li D, Manjula BN, Acharya SA. 2008. Autoxidation of the site-specifically PEGylated hemoglobins: role of the PEG chains and the sites of PEGylation in the autoxidation. Biochemistry. 47:10981–10990.
- Hu T, Li D, Manjula BN, Brenowitz M, Prabhakaran M, Acharya SA. 2009. PEGylation of Val-1(alpha) destabilizes the tetrameric structure of hemoglobin. Biochemistry. 48:608–616.
- Hu T, Li D, Meng F, Prabhakaran M, Acharya SA. 2011. Increased inter dimeric interaction of oxy hemoglobin is necessary for attenuation of reductive pegylation promoted dissociation of tetramer. Artif Cells Blood Substit Immobil Biotechnol. 39:69–78.
- Hu T, Manjula BN, Li D, Brenowitz M, Acharya SA. 2007. Influence of intramolecular cross-links on the molecular, structural and functional properties of PEGylated haemoglobin. Biochem J. 402: 143–151.
- Hu T, Prabhakaran M, Acharya SA, Manjula BN. 2005. Influence of the chemistry of conjugation of poly(ethylene glycol) to Hb on the oxygen-binding and solution properties of the PEG-Hb conjugate. Biochem J. 392:555–564.
- Huang ZF, Ye CH, Liu ZJ, Wang XJ, Chen HB, Liu YL, et al. 2012. Solid-phase N-terminus PEGylation of recombinant human fibroblast growth factor 2 on heparin-sepharose column. Bioconjugate Chem. 23:740–750.
- Iafelice R, Cristoni S, Caccia D, Russo R, Rossi-Bernardi L, Lowe KC, Perrella M. 2007. Identification of the sites of deoxyhaemoglobin PEGylation. Biochem J. 403:189–196.
- Jahr JS, Akha AS, Holtby RJ. 2012. Crosslinked, polymerized, and PEG-conjugated hemoglobin-based oxygen carriers: clinical safety and efficacy of recent and current products. Curr Drug Discovery Technol. 9:158–165.
- Kellett GL. 1971. Dissociation of hemoglobin into subunits. Ligand-linked dissociation at neutral pH. J Mol Biol. 59:401–424.
- Kelly SM, Price NC. 2000. The use of circular dichroism in the investigation of protein structure and function. Curr Protein Pept Sci. 1:349–384.
- Li C, Lin Q, Wang J, Shen L, Ma G, Su Z, Hu T. 2012. Preparation, structural analysis and bioactivity of ribonuclease A-albumin conjugate: tetra-conjugation or PEG as the linker. J Biotechnol. 162:283–288.
- Li D, Hu T, Manjula BN, Acharya SA. 2009. Extension arm facilitated pegylation of alphaalpha-hemoglobin with modifications targeted exclusively to amino groups: functional and structural advantages of free Cys-93(beta) in the PEG-Hb adduct. Bioconjugate Chem. 20:2062–2070.
- Lu XL, Zhao DX, Su ZG. 2004. Purification of hemoglobin by ion exchange chromatography in flow-through mode with PEG as an escort. Artificial Cells Blood Substitutes and Immobilization. Biotechnology. 32:209–227.
- Lunelli L, Zuliani P, Baldini G. 1994. Evidence of hemoglobin dissociation. Biopolymers. 34:747–757.
- Mu Q, Hu T, Yu J. 2013. Molecular insight into the steric shielding effect of PEG on the conjugated staphylokinase: biochemical characterization and molecular dynamics simulation. PLoS One. 8:e68559.
- Pasut G, Veronese FM. 2012. State of the art in PEGylation: the great versatility achieved after forty years of research. J Control Release. 161:461–472.
- Rameez S, Alosta H, Palmer AF. 2008. Biocompatible and biodegradable polymersome encapsulated hemoglobin: a potential oxygen carrier. Bioconjugate Chem. 19:1025–1032.
- Ruckpaul K, Rein H, Jung F. 1971. Correlations between thermal stability and circular dichroism of hemoglobin derivatives of different species. FEBS Lett. 13:193–194.
- Sakai H, Okuda N, Takeoka S, Tsuchida E. 2011. Increased viscosity of hemoglobin-based oxygen carriers retards NO-binding when perfused through narrow gas-permeable tubes. Microvasc Res. 81:169–176.
- Sakai H, Takeoka S, Yokohama H, Seino Y, Nishide H, Tsuchida E. 1993a. Purification of concentrated hemoglobin using organic-solvent and heat-treatment. Protein Express Purif. 4:563–569.
- Sakai H, Takeoka S, Yokohama H, Seino Y, Nishide H, Tsuchida E. 1993b. Purification of concentrated hemoglobin using organic solvent and heat treatment. Protein Express Purif. 4:563–569.
- Snell SM, Marini MA. 1988. A convenient spectroscopic method for the estimation of hemoglobin concentrations in cell-free solutions. J Biochem Biophys Methods. 17:25–33.
- Tsuchida E, Sou K, Nakagawa A, Sakai H, Komatsu T, Kobayashi K. 2009. Artificial oxygen carriers, hemoglobin vesicles and albumin-hemes, based on bioconjugate chemistry. Bioconjugate Chem. 20:1419–1440.
- Vandegriff KD, Malavalli A, Minn C, Jiang E, Lohman J, Young MA, et al. 2006. Oxidation and haem loss kinetics of poly(ethylene glycol)-conjugated haemoglobin (MP4): dissociation between in vitro and in vivo oxidation rates. Biochem J. 399:463–471.
- Vandegriff KD, Young MA, Lohman J, Bellelli A, Samaja M, Malavalli A, Winslow RM. 2008. CO-MP4, a polyethylene glycol-conjugated haemoglobin derivative and carbon monoxide carrier that reduces myocardial infarct size in rats. Br J Pharmacol. 154: 1649–1661.
- Winslow RM. 2000. Blood substitutes. Adv Drug Deliv Rev. 40: 131–142.
- Winslow RM. 2004. MP4, a new nonvasoactive polyethylene glycol-hemoglobin conjugate. Artif Organs. 28:800–806.
- Xue X, Li D, Yu J, Ma G, Su Z, Hu T. 2013. Phenyl linker- induced dense PEG conformation improves the efficacy of C-terminally monoPEGylated staphylokinase. Biomacromolecules. 14:331–341.