Abstract
Background/Aims: The synthesis of methylene blue (MB) encapsulated in silica nanoparticles (SiNPs) as an application for photodynamic therapy is reported in this study. Semi-rigid tissues with optical properties similar to that of human tissues were used as sample materials to determine the applicability of MB encapsulated in SiNPs. Materials and methods: The changes in optical properties of the tissue treated with encapsulated MB under light exposure (Intensity at 664 nm ∼11.9 mW/cm2) were observed. The optimal exposure time required for naked MB and MB-SiNP to destroy red blood cells (RBCs) in the artificial tissue was also determined. Results: The comparative analysis between the results of applying naked MB and MB encapsulated in SiNPs in the treatment of artificial tissue confirmed that the encapsulated MB is 62 percent higher in efficacy than naked MB. The results established the applicability of MB encapsulated in SiNP on artificial tissue and possible application on human tissue.
Introduction
Photodynamic therapy (PDT) has been put forward as a new medical application in the treatment of cancer. This method is based on the transmission of photosensitizers (PS) to the target tissue under light exposure (CitationHe et al. 2009). Singlet oxygen (1O2) produced during the exposure will destroy the cells via cytotoxicity (CitationHuang 2005). However, the efficacy of 1O2 production decreases drastically due to the lower amount of PS that reaches the projected target. The decrease in PS occurs as a result of the defense mechanism enhanced by the reticulo-endothelial system (RES), or the destruction of PS by the macrophage system in the body. However, macrophages usually ignore foreign objects that are biocompatible and of a size less than 100 nm (CitationHermes and Leuschner 2005). Thus, the problems of efficacy associated with engulfment by macrophages can be solved using the nanoparticle as a medium of transport. Silica nanoparticles (SiNPs) can be one such medium, as encapsulating carriers of PS. The unique properties in SiNPs, such as low toxicity and high biocompatibility, make them ideal candidates as encapsulation material for a drug delivery system (DDS) (CitationBharali et al. 2005). Moreover, they are easily synthesized, with low polydispersity at low temperatures, the tendency for biomolecular compounds to agglutinate to their outer surface, and the ability of their inner surface to encapsulate the PS (CitationKneuer et al. 2000, CitationZhao et al. 2004, CitationSantra et al. 2004).
Methylene blue (MB) was selected as a standard material for PDT application (used as a PS in our study). In the naked form, MB exhibits low dark toxicity and generates high 1O2 when applied in PDT (CitationRedmond and Gamlin 1999). Thus, this study investigated the effects of the MB encapsulated by SiNPs on RBCs, using artificial tissue (light absorption component) as test sample. The properties measured comprised transmittance, reflectance, and optimal exposure time. The changes in absorption, scattering coefficient, the relation between the depth and the transmittance, and the Half Value Layer (HVL) of the RBCs were also analyzed. Afterwards, the efficacy of encapsulated MB and naked MB in RBC destruction were comparatively analyzed.
Materials and methods
SiNPs were first prepared using a microemulsion method (CitationChatterjee 2008). First, 0.1 ml of ammonia (Sigma Aldrich Cat No: 1336, 21, 6), 200 ml distilled water, and 5.5 g of Tween 80 (Sigma Aldrich Cat. No. P1754) were mixed. The resultant solution was stirred continuously for 15 min. The pH value was measured to be more than 9.0. Next, 6 ml of 1-butanol (Sigma Aldrich Cat No: B8, 590, 0) was added to the solution and stirred for a further 5 min at room temperature. To encapsulate MB, 15 ml of 62.5 mM of MB was added after mixing the 1-butanol in the previous step. After adding the MB, the sample was wrapped in aluminum foil, because of its high sensitivity to light. After 1 h, 2 ml of triethoxyvinylsilane (TEVS) (Sigma Aldrich Cat. No. 175560) was added, and the resultant solution was constantly stirred at a temperature of 27°C for 20 h. After completing the procedure, dialysis was performed on the solution using a dialysis membrane (after soaking the dialysis membrane in distilled water for 5 min) (CitationChatterjee 2008). Details on steps of loading MB in the SiNPs and the calculation of MB-loading efficiency were discussed in our previous work (CitationMakhadmeh et al. 2014).
One hundred ml of artificial tissue was prepared by dissolving 2 g of agar (No. 9405, Sigma Aldrich) in 40 ml of distilled water and 40 ml of phosphate buffered saline (PBS) (1 tablet in 200 ml distilled water, No. P4417, Sigma Aldrich). The solution was heated up to 100°C for 3 min. The sample was stirred during the heating and cooling stages. After the solution had cooled down to below 80°C, 3 g of 5–10 μm diameter silica powder was added to mimic biological tissue scattering (CitationWagnières et al. 1997). The solution was subsequently placed in a sonicator for 5 min. Next, 1 ml of solution ink (Abs. equal to 0.18 at 500 nm after diluting 100-fold), 20 ml of bovine serum, and 0.2 ml of intralipid (used to mimic biological tissue scattering, SIGMA-ALDRICH, L141, 117k07251) (CitationWagnières et al. 1997) were included into the solution at 45°C. In addition, 1.5 ml of diluted blood (Abs. equal to 0.90 at 500 nm after diluting 100-fold), MB encapsulated by SiNPs with a final concentration of 2.58 μM, and 1 ml of penicillin were added at 40°C.
The final solution was placed in between two glass slides with partitions roughly equal to 0.71 mm (sample thickness). Thereafter, the solution was stored all night under 4°C. The following day, 5 sample slices were subjected to light exposure (Intensity at 664 nm ∼11.9 mW/cm2 from an arc lamp at a distance of 30 cm away) at different exposure times (0, 10, 25, 45, and 70 min). The transmittance T and reflectance R spectra were measured in the samples with the use of the integrating sphere in a NIR-UV-VIS spectrophotometer. Based on the Kubelka-Munk theory (CitationJaradat et al. 2011), the scattering and absorption coefficients of the spectra were derived. The parameters calculated or measured include the relation between the thickness of the sample and the transmittance, the thickness of the material at which the intensity decreased by one half (HVL), and the optimal exposure time that is required to eliminate half of the RBCs. An identical measurement series was also conducted on naked MB for comparison.
Results
Effect of naked and encapsulated MB on the optical properties of artificial tissue
In our previous work, images of SiNPs taken from Transmission Electron Microscopy (TEM) showed that the SiNPs were generally spherical with an average size of about 8 nm (CitationMakhadmeh et al. 2014). The effect of light source at different exposure times on transmittance of the artificial tissue without MB treatment is as shown in . No significant interaction between SiNPs and RBCs without light was observed, because the maximum absorption peak of the RBCs did not decrease during the overnight storage under refrigeration at 4°C. Furthermore, no significant change in the transmittance spectrum at the critical wavelength (577 nm) was observed.
Figure 1. The changes in the transmittance spectrum of the artificial tissue at different exposure times with neither naked nor encapsulated MB.
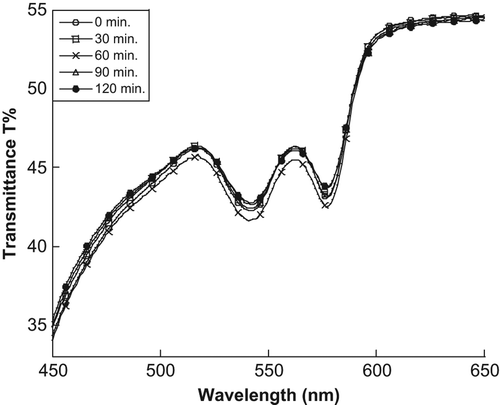
To determine the efficacy of encapsulation, the effects of using encapsulated MB and naked MB were studied. and show changes in the minimum point of the reflectance spectrum when exposed to light, for samples treated with encapsulated MB and naked MB respectively. For the treated samples, an increase is observed at the minimum point of 577 nm (maximum peak of RBC absorption).
Figure 2. The changes in reflectance spectrums of the artificial tissue: (a) treated by encapsulated MB (b) treated by naked MB.
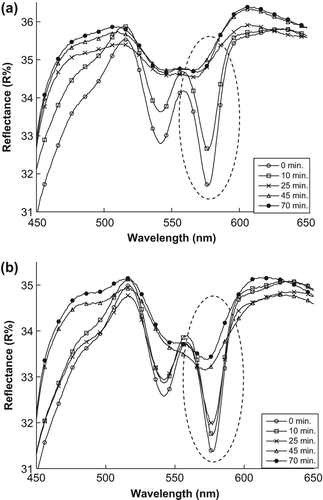
The minimum point of transmittance spectrum for samples treated with encapsulated MB and naked MB shifts, as shown in and , respectively. The minimum point (at 577 nm) increased during a different exposure time because the MB (naked or encapsulated) produced the singlet oxygen, after absorbing enough energy from the light, which destroyed the RBC membranes by singlet oxygen cytotoxicity in the sample and spread the hemoglobin in the sample, thus increasing the transmittance value.
Figure 3. The changes in transmittance spectra of the artificial tissue: (a) treated by encapsulated MB (b) treated by naked MB.
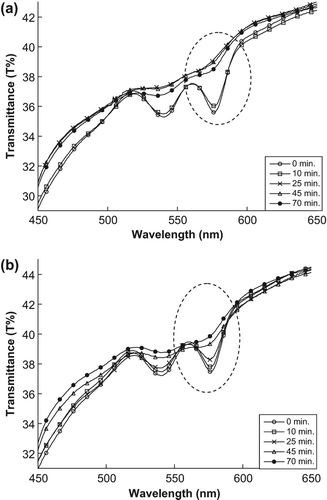
This phenomenon is also observable in the scattering values at 577 nm, as shown in and . This increase in the scattering values at 577 nm leads to the singlet oxygen that is produced after MB absorbs enough light energy, thus destroying the RBC membranes in the sample and then decreasing the absorption and increasing the scattering values.
Figure 4. The changes in scattering spectra of the artificial tissue: (a) treated with encapsulated MB (b) treated with naked MB.

However, the situation is different for the absorption values, as shown in and . Instead, a decrease in the maximum peak of the tissue spectrum of absorption coefficient is observed. This is because the singlet oxygen produced after MB absorbs sufficient light energy destroyed the RBC membrane, resulting in the release of hemoglobin in the sample, which decreases the absorption peak.
Measurement of optimal exposure time
In this part of the study, the optimal exposure time required for the samples (RBCs) to reach the half life time, t50, was measured. shows a decrease of K's maximum peak of the samples with naked and encapsulated MB after exposure to the light source.
Figure 6. (a) Maximum peak of K versus exposure time (b) Optimal exposure time at 50% of fractional hemolysis.
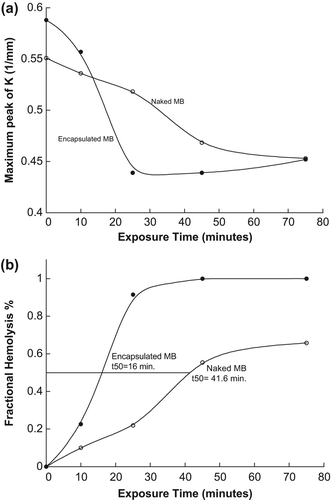
The half-life time of RBCs (when fractional hemolysis reaches 50%) was estimated to be at 16.0 and 41.6 min for samples treated by naked and encapsulated MB respectively, as shown in . This is because the encapsulated MB molecules were agglomerated by the SiNP membranes, thus increasing the absorbance of light energy and singlet oxygen. However, for naked MB molecules, the molecules are well distributed in the sample, which causes a slower interaction with light.
Relation between transmittance and the thickness of artificial tissue
The changes in transmittance with increase in thickness (D) of the sample were measured for five samples at 577 nm, as shown in . The HVL of the samples was also measured, and found to be 0.67 and 0.70 mm for samples treated with encapsulated and naked MB respectively. The related equation between T and D for the samples was derived from the logarithmic relation as shown in .
Discussions
The results from mean that there was no effect of the light source on the RBCs in the artificial tissue without the photosensitizer (MB). In addition, the SiNP membranes exhibited no effects of cytotoxicity because the RBCs were not destroyed after keeping the sample in the refrigerator overnight. The reason for the increasing reflectance value in can be attributed to the encapsulated and naked MB that absorbs the light energy and produces 1O2 which destroys the membranes of the RBCs in the tissue, leading to spread of the hemoglobin. The changes in the hemoglobin were determined using data on the RBCs’ absorption peaks. As such, MB and its interaction with light is mostly responsible for the changes in the transmittance, scattering, and absorption spectra observed.
The effects of encapsulated and naked MB on RBCs, as shown in , proved that the encapsulated MB destroyed RBCs faster than naked MB because the encapsulated MB molecules were clustered together by the SiNP membranes, causing more absorbance of light energy and more singlet oxygen production compared to naked MB which spread in the sample. indicates that the decrease in the absorption coefficient values leads to MB that absorbs enough light energy and produces 1O2, which is the cytotoxic agent used to destroy RBCs in the artificial tissue. No significant change between the samples treated with encapsulated MB and those with naked MB was observed after comparatively analyzing the values of HVL for both samples as shown in . The results further showed that the relationship between T and D can be used to measure the HVL for other samples without having to replicate experiments.
It can be concluded from the results that encapsulation greatly improves the efficiency and ability of MB as a photodynamic agent in the treatment of artificial tissues, compared to the naked MB. As shown in the in vitro studies above, encapsulated MB causes more damage to RBCs compared to naked MB. SiNPs demonstrated a high efficacy of 62% of photo-cytotoxicity (percentage between t50 for naked and encapsulated MB) and faster interactions compared to naked MB at this efficacy percentage.
Acknowledgments
The author/s would like to acknowledge the support from staff at the School of Physics and Natori Lab@INFORMM, USM.
Declaration of interest
The authors report no declarations of interest. The authors alone are responsible for the content and writing of the paper.
This work has been supported by the USM RU-PRGS grant (1001/PFIZIK/846087) and the USM RU grant (1001/PFIZIK/814113).
References
- Bharali DJ, Klejbor I, Stachowiak EK, Dutta P, Roy I, Kaur N, et al. 2005. Organically modified silica nanoparticles: A nonviral vector for in vivo gene delivery and expression in the brain. Proc Natl Acad Sci U S A. 102:11539–11544.
- Chatterjee DK, Fong LS, Zhang Y. 2008. Nanoparticles in photodynamic therapy: An emerging paradigm. Adv Drug Deliv Rev. 60:1627–1637.
- He X, Wu X, Wang K, Shi B, Hai L. 2009. Methylene blue-encapsulated phosphonate-terminated silica nanoparticles for simultaneous in vivo imaging and photodynamic therapy. Biomaterials. 30:5601–5609.
- Hermes J, Leuschner C. 2005. Nanofabrication towards biomedical applications. Wiley-VCH:Weinheim.
- Huang Z. 2005. A review of progress in clinical photodynamic therapy. Technol Cancer Res Treat. 4:283–293.
- Jaradat A, Al-Akhras M-AH, Makhadmeh G, Aljarrah K, Al-Omari A, Ababneh Z, et al. 2011. Artificial semi-rigid tissue sensitized with natural pigments: Effect of photon radiations. J Pharm Bioallied Sci. 3:266–276.
- Kneuer C, Sameti M, Haltner EG, Schiestel T, Schirra H, Schmidt H, Lehr C-M. 2000. Silica nanoparticles modified with aminosilanes as carriers for plasmid DNA. Int J Pharm. 196:257–261.
- Makhadmeh GN, Aziz AA, Razak KA. 2014. Loading and unloading properties of encapsulated methylene blue in silica nanoparticles for photodynamic applications. Adv Mater Res. 1024:292–295.
- Redmond RW, Gamlin JN. 1999. A compilation of singlet oxygen yields from biologically relevant molecules. Photochem Photobiol. 70:391–475.
- Santra S, Yang H, Dutta D, Stanley JT, Holloway PH, Tan W, et al. 2004. TAT conjugated, FITC doped silica nanoparticles for bioimaging applications. Chem Commun.(24):2810–2811.
- Wagnières G, Cheng S, Zellweger M, Utke N, Braichotte D, Ballini J-P, van den Bergh H. 1997. An optical phantom with tissue-like properties in the visible for use in PDT and fluorescence spectroscopy. Phys Med Biol. 42:1415–1426.
- Zhao Y, Sadtler B, Lin M, Hockerman GH, Wei A. 2004. Nanoprobe implantation into mammalian cells by cationic transfection. Chem Commun.(7):784–785.