ABSTRACT
Three ordered mesoporous silicas (OMSs) with different pore sizes and pore architectures were prepared and modified with amine functional groups by a postgrafting method. The carbon dioxide (CO2) adsorption on these amine-modified OMSs was measured by using microbalances at 348 K, and their adsorption capacities were found to be 0.2–1.4 mmol g−1 under ambient pressure using dry 15% CO2. It was found experimentally that the CO2 adsorption capacity and adsorption rate were attributed to the density of amine groups and pore volume, respectively. A simple method is described for the production of densely anchored amine groups on a solid adsorbent invoking direct incorporation of tetraethylenepentamine onto the as-synthesized OMSs. Unlike conventional amine-modified OMSs, which typically show CO2 adsorption capacity less than 2 mmol g−1, such organic template occluded amine-OMS composites possessed remarkably high CO2 uptake of approximately 4.6 mmol g−1 at 348 K and 1 atm for a dry 15% CO2/nitrogen feed mixture. The enhancement of 8% in CO2 adsorption capacity was also observed in the presence of 10.6% water vapor. Durability tests done by cyclic adsorption-desorption revealed that these adsorbents also possess excellent stability.
Current available CO2 separation schemes for fossil fuel combustion normally invoke absorption by a liquid and are generally limited by the high capital and operation costs. Amine-modified solid sorbents are attractive for CO2 capture from flue gas streams because of the low energy consumption of the regeneration process. In this study, an easy method is developed by directly introducing tetraethylenepentamine onto the as-synthesized OMSs, which represents not only a time-saving route but also superior CO2 adsorption capacities and durability after repeated adsorption-desorption cycles, revealing some opportunities for future practical applications.
INTRODUCTION
Global warming caused by a progressive increase of carbon dioxide (CO2) in the atmosphere has becoming an important and challenging issue.Citation1,Citation2 In particular, research and development invoking cost-effective technologies for separation and capture of CO2 is one of the most crucial tasks in carbon sequestration. Current available CO2 separation processes, limited by the high capital and operation costs, generally use absorption by a liquid, adsorption by a solid, or separation by selective transport through a membrane.Citation3 The high costs for these schemes are mostly due to low mass fluxes in the separation units, production of high-pressure steam, and high energy consumption during regeneration of the adsorbents. As a result, crucial issues such as cost, operation durability, selectivity of the separation agents, and complexity of the process must be considered.
The majority of the commercial CO2 capture processes use technologies based on chemical absorption by alkanolamine solvents,Citation4–8 including the primary (e.g., monoethanolamine), secondary (e.g., diethanolamine), and tertiary (e.g., methyldiethanolamine) amines. However, because the formation of carbamate ions, known to arise by rapid reaction with CO2, is normally associated with a relatively high heat of absorption, the cost of regenerating primary and secondary amines is high. Tertiary amines lack the N–H bond required to form the carbamate ion and hence do not react directly with CO2. However, in aqueous solutions, tertiary amines assist in the hydrolysis of CO2 to form bicarbonate and protonated amines. Nevertheless, apart from high energy consumption, such liquid amine-based CO2 separation processes also have severe drawbacks, including solvent deterioration, equipment corrosion, and limited amine concentration in the aqueous phase because of viscosity and foaming issues. Although several new processes such as absorption/adsorption by solid absorbents/adsorbents and membrane and cryogenic separation have been developed to handle these problems, most of the new processes are still far from practical industrial applications.
Over the past few years, extensive studies have been conducted to investigate methods of physisorption using nanoporous solids for sequestration of CO2, for example, microporous zeolites,Citation9–14 porous carbon materials,Citation15,Citation16 porous coordination polymers, or organic nanostructures.Citation17,Citation18 Among them, alkali ion-exchanged faujasite (X and Y) zeolites and high surface area-activated carbon materials have CO2 uptake capacities up to 10–13 mmol g−1. However, other materials exhibit low CO2 adsorption capacity, which is typically smaller than the benchmark value of approximately 2 mmol g−1 adsorbent for practical commercialization. In general, these materials are inclined to have problems such as low capacity, poor selectivity, poor tolerance to water, and high-temperature regeneration or activation. Some studies have revealed that metal-organic frameworksCitation19,Citation20 and van der Waals crystalsCitation21 are potential CO2 adsorbents.
Ordered mesoporous silicas (OMSs) are intensively attractive for applications as gas adsorbents because of their unique properties including tunable pore size (2–50 nm), narrow pore size distribution, high surface area, large pore volume, and good thermal stability. The pore surfaces of OMSs are abundant with hydroxyl groups, which favored grafting of organic functional groups. In terms of CO2 capture, the most interesting organic functional groups would be polyamine compounds that possess high amine group density (a large amount of CO2 sorption sites) and slow CO2 adsorption/desorption kinetics. Therefore, amine-modified OMSs provide concrete objective function to serve as the CO2 “molecular basket” adsorbents, which facilitates a synergic effect on the CO2 adsorption capacity and adsorption kinetics between nanoporous supports and polyamines (especially at high polyamine loading). For the polyethylenimine (PEI)-impregnated MCM-41, a high CO2 adsorption capacity of 246 mg g−1 PEI, which is 30 times higher than that of MCM-41 and is ∼2.3 times that of the undiluted PEI, was obtained with a PEI loading of approximately 50 wt %.Citation22,Citation23 Some other different types of polyamines grafted on various OMSs, such as MCM-41, MCM-48, and SBA-15 have been examined for CO2 adsorption.Citation24–34 Nonetheless, their CO2 adsorption capacities were normally below the benchmark value of 2 mmol g−1. Sayari and co-workersCitation35–37 Citation Citation37 incorporated amines into the pore-expanded MCM-41, which was capable of “scrubbing” CO2 in greater quantity of amine and was more resistant to moisture compared with the other supports including activated carbon, silica gel, and standard MCM-41 silica. Repeated adsorption-desorption cycles indicated that these novel adsorbents exhibited good cyclic stabilities. Hicks et al.Citation38 fabricated a covalently tethered hyperbranched aminosilica adsorbent, which is capable of adsorbing CO2 reversibly with a high capacity of 3.1 mmol g−1 at 298 K and multicycle stability. Recently, Lu et al.Citation39 synthesized amine-modified mesoporous spherical silica particles, which are efficient for CO2 capture, and their adsorption performance can be maintained during 16 cycles of adsorption and thermal regeneration.
From the literature citations mentioned above, the effect of different amine-supported silica on the CO2 adsorption was extensively investigated. However, studies concerning the influence of pore structure of silica support and amine-grafting methods on CO2 adsorption capacities are lacking. In this study, three different pore architectures of mesoporous silicas and two different grafting methods have been adopted to prepare aminemodified adsorbent materials (as shown in ), namely, (1) by incorporating 3-[2-(2-aminoethylamino) ethylamino]propyltrimethoxysilane (AEPTMS) onto mesoporous silica SBA-15, KIT-6, and mesocellular silica foam (MSF) and (2) by directly incorporating tetraethylenepentamine (TEPA) onto the as-synthesized SBA-15 without removing the organic templates. These aminefunctionalized porous materials were characterized by a variety of different analytical and spectroscopic techniques, including nitrogen (N2) adsorption/desorption, X-ray diffraction (XRD), elemental analysis (EA), Fourier-transformed IR (FTIR), and thermogravimetric analysis (TGA). Among them, the latter method not only represents a time-saving route but also produces adsorbents with superior CO2 adsorption capacities and durability after repeated adsorption-desorption cycles, revealing some opportunities for future practical applications.
MATERIALS AND METHODS
Materials Preparation
The parent SBA-15, KIT-6, and MSF samples were synthesized according to the methods reported in the literature.Citation40–42 In a typical synthesis of SBA-15 samples, 2.9 g of neutral tri-block copolymer surfactant (Pluronic 123, EO20PO70EO20, molecular weight = 5800; Aldrich) was dissolved in a mixture of 37% HCl solution (12.2 g) and water (84.7 g) at room temperature. After addition of tetraethyl orthosilicate (TEOS) (98%; ACROS), the resultant mixture was stirred at 313 K for 20 hr and then transferred into a polypropylene bottle and reacted at 373 K under static condition for 24 hr. To fabricate KIT-6 samples, 2 g of Pluronic 123 was dissolved in 77 g of water and 3.9 g of 35 wt % HCl solution with stirring at 308 K. After complete dissolution, 2 g of BuOH (98%; ACROS) was added at once. After 1 hr of stirring, 4.3 g of TEOS was added to the homogeneous clear solution. This mixture is kept under vigorous stirring at 308 K for 24 hr followed by aging at 373 K for 24 hr. For the preparation of MSF samples, 2 g of Pluronic 123 was dissolved in 75 ml of aqueous 1.6 N HCl at room temperature, then 12 mg of NH4F (ACROS) and 1.5 g of trimethylbenzene (98%; ACROS) were added into the mixture. After stirring for 1 hr at 313 K, 4.3 g of TEOS was added to the mixture. The resulting reaction mixture was stirred at 313 K for 20 hr followed by aging at 373 K for 24 hr. The solid products of as-synthesized SBA-15, KIT-6, and MSF were recovered by filtration and dried at room temperature overnight followed by removal of organic template by calcination at 823 K. AEPTMS-modified SBA-15, KIT-6, and MSF materials were prepared by a postgrafting method. Typically, calcined SBA-15, KIT-6, and MSF (0.5 g) were first dried at 398 K for 6 hr in air and then were refluxed in toluene solution (24 ml) of AEPTMS (0.42 ml) at 383 K for 24 hr under an N2 flow. The products (denoted as AEPTMS-SBA-15L, AEPTMS-KIT-6L, and AEPTMS-MSF-L, respectively) were washed with toluene and dried at 333 K overnight. The same procedure was followed except that 4.2 ml of AEPTMS was used. Thus, the samples named AEPTMSSBA-15H, AEPTMS-KIT-6H, and AEPTMS-MSF-H, respectively can be obtained.
To incorporate TEPA onto the as-synthesized SBA-15 materials (i.e., in the presence of organic templates; denoted as SBA-15as), certain amounts of TEPA were dissolved in 25 g of ethanol under stirring for 0.5 hr, and then 0.5 g of SBA-15as was added into the solution. After stirring and refluxing for 2 hr, the mixture was evaporated at 353 K, followed by drying at 373 K for 1 hr. The products were obtained (denoted as TEPA-SBA-15as-x, where x represents the amount of N in wt %) by filtration, washing with water, and then air-drying at room temperature.
Characterization Methods
XRD patterns were recorded on a PANalytical (X'Pert PRO) instrument using Cu K α radiation (λ= 0.1541 nm). EAs were performed using a CHN elemental analyzer (Heraeus VarioIII). Nitrogen adsorption/desorption isotherms were measured at 77 K on a Micromeritics ASAP 2010 volumetric adsorption analyzer. FTIR spectra were collected on a Bio-Rad 165 spectrometer with 4 cm−1 resolution using KBr pellets at room temperature.
CO2 Adsorption Capacity Measurements
To determine the adsorption and desorption properties of various adsorbents, a modified thermogravimetric analyzer (Pyris 6; PerkinElmer) with a H2O saturator is shown schematically in . In a typical adsorption/desorption process, approximately 10 mg of adsorbent placed in a sample cell was heated to 373 K under N2 flow (50 ml min−1) and then was maintained at that temperature for at least 30 min until no weight loss was observed. Subsequently, the sample was cooled down to 348 K, and 15% dry CO2 was introduced at a flow rate of 50 ml min−1. After adsorption, the gas was switched to pure N2 flow (50 ml min−1) to advance the desorption procedure at the same temperature. The time required for each adsorption and desorption cycle was 120 min. The sensitivity and accuracy of TGA microbalance are 10 μg and 0.1%, respectively. The influence of moisture on CO2 adsorption capacities was also investigated together with cyclic adsorption/desorption measurements to assess the stability of the adsorbents.
RESULTS AND DISCUSSION
The small-angle XRD patterns of the pristine and AEPTMS-modified SBA-15 samples are shown in . The pristine SBA-15 exhibited one main intensive (100) peak at 2θ of approximately 0.9 ° and two weak (110) and (200) diffraction peaks, indicating the existence of well-ordered hexagonal arrays and two-dimensional channel structure. In , an intense peak and a weak shoulder in the small-angle region can be indexed as (211) and (220) planes of three-dimensional cubic structure, which are the characteristic features of KIT-6. The d spacing ratio of these two features is exactly 0.867, which implies that KIT-6 samples with mesoporous bicontinuous cubic space group Ia3d.Citation43 Likewise, the pore uniformity of MSF was also confirmed by the XRD peak at 2θ of approximately 0.5 ° (). However, upon incorporating AEPTMS into the pristine matrix, notable decreases in diffraction peak intensities were observed. As displayed in , all N2 adsorption/desorption curves of the pristine SBA-15, KIT-6, and MSF samples showed typical type IV isotherms with a well-defined hysteresis loop, revealing the existence of ordered mesopores in the frameworks, in accordance with XRD results. Nonetheless, the diminishing of hysteresis loops on incorporating a substantial amount of AEPTMS functional groups in the AEPTMSSBA-15H and AEPTMS-KIT-6H samples may be attributed to the blockage of mesopore channels. As shown in , notable decreases in total pore volume (V), Brunauer-Emmet-Teller surface area (S), and Barrett-Joyner-Halenda pore size (D) were observed for AEPTMS-modified samples compared with the pristine SBA-15, KIT-6, and MSF samples. It should be noted that the MSF sample possesses a much larger pore volume (2.71 cm3 g−1) and pore size (22 nm) than the pristine SBA-15 (V = 1.36 cm3 g−1; D = 10 nm) and KIT-6 (V = 1.01 cm3 g−1; D = 8 nm), some mesoporosities remained accessible even after a considerable amount of AEPTMS was loaded onto the sample, as revealed by the existence of hysteresis loop in the isotherm of AEPTMS-MSF-H sample (). The presence of AEPTMS functional groups in the surface-modified SBA-15, KIT-6, and MSF samples was confirmed by FTIR spectroscopy (data not shown). Compared with their pristine samples, the bands at 1590 and 1475 cm−1 are attributed to the NH2 scissoring vibration and CH stretching vibration in the amine functional groups, respectively, were evident for AEPTMS-modified SBA-15, KIT-6, and MSF samples. Further measurements of N2 contents by EA were found to vary from 3.2 to 10.1 wt % in the AEPTMS-modified SBA-15, KIT-6, and MSF samples ().
Table 1. Physicochemical properties and CO2 adsorption performances of parent and AEPTMS-modified SBA-15, KIT-6, MSF, as-synthesized SBA-15, and TEPA-modified SBA-15as samples
Figure 3. Small-angle XRD patterns and N2 adsorption-desorption isotherms of pure and AEPTMS-modified mesoporous silica SBA-15 (a), KIT-6 (b), and MSF (c).
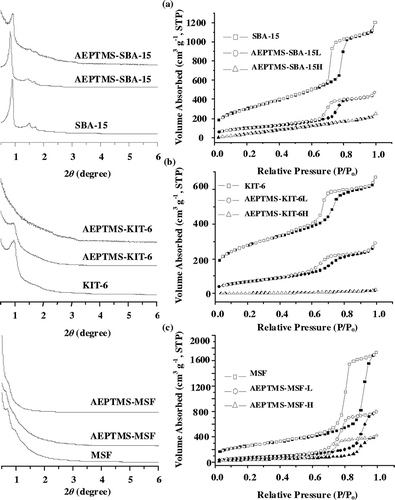
The CO2 adsorption capacities of various AEPTMS-modified SBA-15, KIT-6, and MSF are summarized in . Unlike the pristine SBA-15, KIT-6, and MSF samples, which showed almost no CO2 uptake, AEPTMS-modified samples indicated the adsorption capacities of approximately 0.2–1.4 mmol g−1. Among them, total amounts of adsorbed CO2 for AEPTMS-SBA-15H, AEPTMS-KIT-6H, and AEPTMS-MSF-H samples were 1, 1.1, and 1.4 mmol g−1, respectively, which showed higher amine efficiencies (CO2/N) and CO2 adsorption capacities than those for AEPTMS-SBA-15L, AEPTMS-KIT-6L, and AEPTMS-MSF-L samples. The correlation of CO2 adsorption capacity with surface density of amine is shown in . An obvious correlation between the CO2 adsorption capacity and surface density of amine of the amine-functionalized adsorbents could be found. The chemical adsorption pathway of amine active sites and CO2 is based on the formation of ammonium carbamate in anhydrous conditions on the amine-modified silicas, as shown below:
Figure 4. Correlation of surface density of amine with CO2 adsorption capacity for various AEPTMS-modified mesoporous silicas.
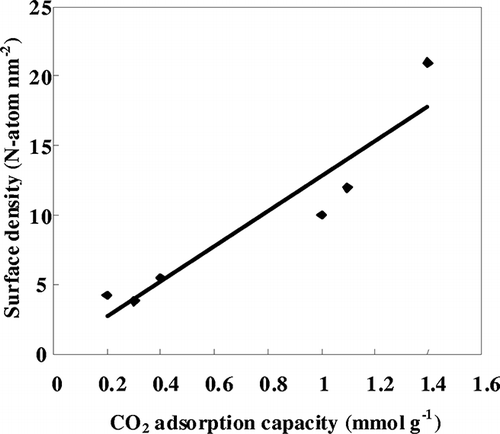
It is indicated that the isolated amine groups are ineffective in CO2 capture because of the stoichiometric CO2/N ratio of 0.5.
The CO2 uptake rate of AEPTMS-SBA-15H, AEPTMS-KIT-6H, and AEPTMS-MSF-H samples is shown in . The two-dimensional pore architecture of AEPTMSMSF-H and AEPTMS-SBA-15H samples with larger pore volume results in a higher CO2 adsorption rate. As evidenced by N2 adsorption/desorption measurements, however, three-dimensional architecture pores of AEPTMS-KIT-6H sample were partially filled by AEPTMS and a certain number of pores may be blocked, leading to the lower CO2 uptake rate. It is clear that the CO2 uptake rate is in proportion with the pore volume of aminefunctionalized adsorbents, suggesting that the pore architecture of aminosilicas may not be the parameter influencing the CO2 adsorption rate.
To increase surface density of amine on silica support, incorporation of different amounts of TEPA into the as-synthesized SBA-15as was performed. The small-angle XRD patterns in revealed that the structure of SBA-15as remained practically intact. However, upon increasing the contents of TEPA, the peak intensity decreases and the higher order (110) and (200) diffractions are less resolved. Furthermore, no peaks in the TEPA-SBA-15as-16.7 sample were observed, which is due to the fact that mesoporous structures of as-synthesized SBA-15 may be distorted by TEPA. In addition, progressive decreases in N2 adsorption amounts and hysteresis loops () were evident upon increasing TEPA loading. The FTIR spectra in also revealed the presence of amine compounds in the TEPA-SBA-15as-x samples, as evidenced by the absorption bands near 1590 cm−1. Further quantitative measurements by elemental analysis showed that the N2 contents in these samples range from 2.6 to 16.7 wt %, as shown in .
Figure 6. (a) XRD patterns, (b) N2 adsorption-desorption isotherms, and (c) FTIR spectra of TEPA-SBA-15as samples.
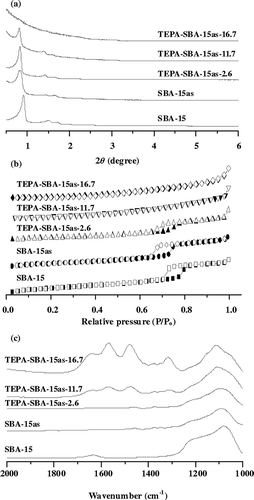
The effects of the TEPA loading amount on CO2 ad sorption capacity and amine efficiency (CO2/N) of various TEPA-SBA-15as-x absorbents are summarized in . It is obvious that both CO2 adsorption capacity and amine efficiency (CO2/N) of the composite increase with increasing amount of TEPA loaded on as-synthesized SBA-15. Again, this result may be attributed to the increase in surface density of amine on TEPA-SBA-15as-x () with increasing N content (x). It is indicative that densely anchored aminosilanes would be more effective as an adsorption site than those isolated on bare silica supports. As a result, the TEPA-SBA-15as-16.7 sample exhibited a remarkably amine efficiency of 0.39 mmol/mmol and high CO2 adsorption capacity of 4.6 mmol g−1, superior to the benchmark value for commercialization (2 mmol g−1).
Figure 7. Variations of CO2 adsorption capacity with surface density of amine observed for various TEPA-SBA-15as samples.
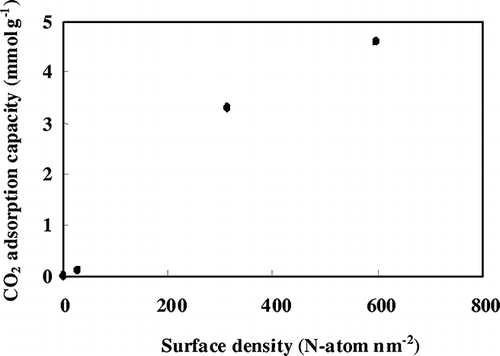
For practical industrial applications in CO2 capture, solid adsorbents should possess not only high adsorptive capacity for CO2 with moisture but also stable cyclic adsorption-desorption performance during long-term operation. The effect of water vapor content in the flue gas on CO2 adsorption of the TEPA-SBA-15as-16.7 sample was examined, as shown in . It was found that as the water vapor content increased from 0 to 10.6%, the CO2 adsorption capacity increased from 4.6 to 5 mmol g−1, which is approximately 9% higher than that obtained from simulated dry flue gas. This may be due to the relatively hydrophobic surface property of the TEPA-SBA-15as adsorbents compared with the pristine SBA-15.Citation44 However, CO2 adsorption capacity slightly decreased from 5 to 4.9 mmol g−1 as the water vapor further increased from 10.6 to 14.1%. This observation may be associated with the competitive adsorption between CO2 and H2O at the same adsorption sites, which is in agreement with the result in the literature.Citation44,Citation45 It should be noted that the CO2 adsorption capacity of TEPA-SBA-15as-16.7 in the 10th adsorption-desorption cycle (i.e., total operation period of approximately 40 hr) () at 348 K under dry 15% CO2 concentration remains at approximately 4.5 mmol g−1, which is still higher than the benchmark value for commercialization (2 mmol g−1). This result is consistent with the recent reports related to the stability of TEPA-grafting mesoporous/microporous silicas on cyclic CO2 adsorption-desorption process.Citation44,Citation46,Citation47
CONCLUSIONS
CO2 uptake measurements on three pore architectures of AEPTMS-modified mesoporous silicas (SBA-15, KIT-6, and MSF), fabricated by a postsynthesis grafting method, indicated their CO2 adsorption capacities of 0.2–1.4 mmol g−1 at 348 K under ambient pressure using dry 15% CO2. The adsorption capacity and rate were found to depend on the surface density of amine groups and pore volume of the mesoporous silicas, respectively. To reach the benchmark value of CO2 adsorption capacity (∼2 mmol g−1) for commercialization, a simple method invoking direct incorporation of TEPA onto the as-synthesized SBA-15 samples in the presence of organic template was developed. Consequently, TEPA-SBA-15as-16.7 absorbent so prepared was found to reach a remarkable CO2 adsorption capacity of approximately 4.6 mmol g−1. This significant enhancement in CO2 uptake compared with the amine-modified silicas without template is ascribed to the increase in surface density of amine. Under the water vapor content of 10.6%, the TEPA-SBA-15as-16.7 sample revealed a further increase in CO2 adsorption capacity to 5 mmol g−1. Furthermore, the absorbent remains stable after repeated adsorption-desorption cycles, revealing a new opportunity for the use in a cyclic adsorption process under humid flue gas conditions.
ACKNOWLEDGMENTS
The financial support of the Taiwan National Science Council (NSC 99-2221-E-151-023-MY2) is gratefully acknowledged.
REFERENCES
- Figueroa , D. , Fout , T. , Plasynski , S. , Mcllvried , H. and Srivastava , R.D. 2008 . Advances in CO2 Capture Technology—the US Department of Energy's Carbon Sequestration Program . Inter. J. Greenhouse Gas Control , 2 : 9 – 20 .
- Zhao , C.W. , Chen , X.P. and Zhao , C.S. 2009 . Effect of Crystal Structure on CO2 Capture Characteristics of Dry Potassium-Based Sorbents . Chemosphere , 75 : 1401 – 1404 .
- Morris , R.E. and Wheatley , P.S. 2008 . Gas Storage in Nanoporous Materials . Angew. Chem. Int. Ed. , 47 : 4966 – 4981 .
- Hook , R.J. 1997 . An Investigation of Some Sterically Hindered Amines as Potential Carbon Dioxide Scrubbing Compounds . Ind. Eng. Chem. Res. , 36 : 1779 – 1790 .
- Veawab , A. , Tontiwachwuthikul , P. and Chakma , A. 1999 . Corrosion Behavior of Carbon Steel in the CO2 Absorption Process Using Aqueous Amine Solutions . Ind. Eng. Chem. Res. , 38 : 3917 – 3924 .
- Rinker , E. , Ashour , S.S. and Sandall , O.C. 2000 . Absorption of Carbon Dioxide into Aqueous Blends of Diethanolamine and Methyldiethanolamine . Ind. Eng. Chem. Res. , 39 : 4346 – 4356 .
- Satyapal , S. , Filburn , T. , Trela , J. and Strange , J. 2001 . Performance and Properties of a Solid Amine Sorbent for Carbon Dioxide Removal in Space Life Support Applications . Energy Fuels , 15 : 250 – 255 .
- Lee , J.Y. , Keener , T.C. and Yang , Y.J. 2009 . Potential Flue Gas Impurities in Carbon Dioxide Streams Separated from Coal-Fired Power Plants . Journal of the Air & Waste Management Association , 59 : 725 – 732 . doi: 10.3155/1047-3289.59.6.725
- Maurin , G. , Bell , R. , Kuchta , B. , Poyet , T. and Llewellyn , P. 2005 . Adsorption of Non Polar and Quadrupolar Gases in Siliceous Faujasite: Molecular Simulations and Experiments . Adsorption , 11 : 331 – 336 .
- Siriwardance , R.V. , Shen , M.S. and Fisher , E.P. 2005 . Adsorption of CO2 on Zeolites at Moderate Temperatures . Energy Fuels , 19 : 1153 – 1159 .
- Walton , K.S. , Abney , M.B. and LeVan , M.D. 2006 . CO2 Adsorption in Y and X Zeolites Modified by Alkali Metal Cation Exchange . Micropor. Mesopor. Mater. , 91 : 78 – 84 .
- Himeno , S. , Tomita , T. , Suzuki , K. and Yoshida , S. 2007 . Characterization and Selectivity for Methane and Carbon Dioxide Adsorption on the All-Silica DD3R Zeolite . Micropor. Mesopor. Mater. , 98 : 62 – 69 .
- Li , P. and Tezel , H. 2007 . Adsorption Separation of N2, O2, CO2 and CH4 Gases by Beta-Zeolite . Micropor. Mesopor. Mater. , 98 : 94 – 101 .
- Pulido , A. , Nachtigall , P. , Zukal , A. , Domínguez , I. and Čejka , J. 2009 . Adsorption of CO2 on Sodium-Exchanged Ferrierites: The Bridged CO2 Complexes Formed between Two Extraframework Cations . J. Phys. Chem. C , 113 : 2928 – 2935 .
- Himeno , S. , Komatsu , T. and Fujita , S. 2005 . High-Pressure Adsorption Equilibria of Methane and Carbon Dioxide on Several Activated Carbons . J. Chem. Eng. Data , 50 : 369 – 376 .
- Hao , G.P. , Li , W.C. , Qian , D. and Lu , A.H. 2010 . Rapid Synthesis of Nitrogen-Doped Porous Carbon Monolith for CO2 Capture . Adv. Mater. , 22 : 853 – 857 .
- Arenillas , A. , Smith , K.M. , Drage , T.C. and Snape , C.E. 2005 . CO2 Capture Using Some Fly ash-Derived Carbon Materials . Fuel , 84 : 2204 – 2210 .
- Navarro , J.A.R. , Barea , E. , Salas , J.M. , Masciocchi , N. , Galli , S. , Sironi , A. , Ania , C.O. and Parra , J.B. 2006 . H2, N2, CO, and CO2 Sorption Properties of a Series of Robust Sodalite-Type Microporous Coordination Polymers . Inorg. Chem. , 45 : 2397 – 2399 .
- Serre , C. , Bourrelly , S. , Vimont , A. , Ramsahye , N.A. , Maurin , G. , Llewellyn , P.L. , Daturi , M. , Filinchuk , Y. , Leynaud , O. , Barnes , P. and Férey , G. 2007 . An Explanation for the Very Large Breathing Effect of a Metal-Organic Framework During CO2 Adsorption . Adv. Mater. , 19 : 2246 – 2251 .
- Walton , K.S. , Millward , A.R. , Dubbeldam , D. , Frost , H. , Low , J.J. , Yaghi , O.M. and Snurr , R.Q. 2008 . Understanding Inflections and Steps in Carbon Dioxide Adsorption Isotherms in Metal-Organic Frameworks . J. Am. Chem. Soc. , 130 : 406 – 407 .
- Serre , C. , Millange , F. , Thouvenot , C. , Nogues , M. , Marsolier , G. , Louer , D. and Férey , G. 2002 . Very Large Breathing Effect in the First Nanoporous Chromium(III)-Based Solids: MIL-53 or CrIII(OH)·{O2C-C6H4-CO2} · {HO2C-C6H4-CO2H}x · H2Oy . J. Am. Chem. Soc. , 124 : 13519 – 13526 .
- Xu , X. , Song , C.S. , Andresen , J.M. , Miller , B.G. and Scaroni , A.W. 2002 . Novel Polyethylenimine-Modified Mesoporous Molecular Sieve of MCM-41 Type as High-Capacity Adsorbent for CO2 Capture . Energy Fuels , 16 : 1463 – 1469 .
- Xu , X. , Song , C.S. , Miller , B.G. and Scaroni , A.W. 2005 . Adsorption Separation of Carbon Dioxide from Flue Gas of Natural Gas-Fired Boiler by a Novel Nanoporous “Molecular Basket” Adsorbent . Fuel Proc. Technol. , 86 : 1457 – 1472 .
- Chang , A.C.C. , Chuang , S.S.C. , Gray , M. and Soong , Y. 2003 . In-Situ Infrared Study of CO2 Adsorption on SBA-15 Grafted with γ-(Aminopropyl)Triethoxysilane . Energy Fuels , 17 : 468 – 473 .
- Gray , M.L. , Soong , Y. , Champagne , K.J. , Pennline , H.W. , Baltrus , J. , Stevens , R.W.J. , Khatri , R.A. , Chuang , S.S.C. and Filburn , T. 2005 . Improved Immobilized Carbon Dioxide Capture Sorbents . Fuel Proc. Technol. , 86 : 1449 – 1455 .
- Hiyoshi , N. , Yogo , K. and Yashima , T. 2005 . Adsorption Characteristics of Carbon Dioxide on Organically Functionalized SBA-15 . Micropor. Mesopor. Mater. , 84 : 357 – 365 .
- Kim , S. , Ida , J. , Guliants , V.V. and Lin , J.Y.S. 2005 . Tailoring Pore Properties of MCM-48 Silica for Selective Adsorption of CO2 . J. Phys. Chem. B , 109 : 6287 – 6293 .
- Zheng , F. , Tran , D.N. , Busche , B.J. , Fryxell , G.E. , Addlemann , R.S. , Zemanian , T.S. and Aardahl , C.L. 2005 . Ethylenediamine-Modified SBA-15 as Regenerable CO2 Sorbent . Ind. Eng. Chem. Res. , 44 : 3099 – 3105 .
- Khatri , R.A. , Chuang , S.S.C. , Soong , Y. and Gray , M. 2006 . Thermal and Chemical Stability of Regenerable Solid Amine Sorbent for CO2 Capture . Energy Fuels , 20 : 1514 – 1520 .
- Knowles , G.P. , Delaney , S.W. and Chaffee , A.L. 2006 . Diethylenetriamine[Propyl(Silyl)]-Functionalized (DT) Mesoporous Silicas as CO2 Adsorbents . Ind. Eng. Chem. Res. , 45 : 2626 – 2633 .
- Yue , M.B. , Chun , Y. , Cao , Y. , Dong , X. and Zhu , J.H. 2006 . CO. 2 Capture by as-Prepared SBA-15 with an Occluded Organic Template . Adv. Funct. Mater. , 16 : 1717 – 1722 .
- Knöfel , C. , Descarpentries , J. , Benzaouia , A. , Zeleňák , V. , Mornet , S. , Llewellyn , P.L. and Hornebecq , V. 2007 . Functionalised Micro-/Mesoporous Silica for the Adsorption of Carbon Dioxide . Micropor. Mesopor. Mater. , 99 : 79 – 85 .
- Liu , X. , Zhou , L. , Fu , Z. , Sun , Y. , Su , W. and Zhou , Y. 2007 . Adsorption and Regeneration Study of the Mesoporous Adsorbent SBA-15 Adapted to the Capture/ Separation of CO2 and CH4 . Chem. Eng. Sci. , 62 : 1101 – 1110 .
- Zeleňák , V. , Badaničová , M. , Halamová , D. , Čejka , J. , Zukal , A. , Murafa , N. and Goerigk , G. 2008 . Amine-Modified Ordered Mesoporous Silica: Effect of Pore Size on Carbon Dioxide Capture . Chem. Eng. J. , 144 : 336 – 342 .
- Reynhardt , J.P.K. , Yang , Y. , Sayari , A. and Alper , H. 2005 . Polyamidoamine Dendrimers Prepared Inside the Channels of Pore-Expanded Periodic Mesoporous Silica . Adv. Funct. Mater. , 15 : 1641 – 1646 .
- Harlick , P.J.E. and Sayari , A. 2007 . Applications of Pore-Expanded Mesoporous Silica. 5. Triamine Grafted Material with Exceptional CO2 Dynamic and Equilibrium Adsorption Performance . Ind. Eng. Chem. Res. , 46 : 446 – 458 .
- Serna-Guerrero , R. , Da'na , E. and Sayari , A. 2008 . New Insights into the Interactions of CO2 with Amine-Functionalized Silica . Ind. Eng. Chem. Res. , 47 : 9406 – 9412 .
- Hicks , J.C. , Drese , J.H. , Fauth , D.J. , Gray , M.L. , Qi , G. and Jones , C.W. 2008 . Designing Adsorbents for CO2 Capture from Flue Gas-Hyperbranched Aminosilicas Capable of Capturing CO2 Reversibly . J. Am. Chem. Soc. , 130 : 2902 – 2903 .
- Lu , C.S. , Bai , H.L. , Su , F.S. , Chen , W.F. , Hwang , J.F. and Lee , H.H. 2010 . Adsorption of Carbon Dioxide from Gas Streams via Mesoporous Spherical-Silica Particles . Journal of the Air & Waste Management Association , 60 : 489 – 496 . doi: 10.3155/1047-3289.60.4.489
- Zhao , D. , Feng , J. , Huo , Q. , Melosh , N. , Fredrickson , G.H. , Chmelka , B.F. and Stucky , G.D. 1998 . Triblock Copolymer Syntheses of Mesoporous Silica with Periodic 50 to 300 Angstrom Pores . Science , 279 : 548 – 552 .
- Lee , J. , Sohn , K. and Hyeon , T. 2001 . Fabrication of Novel Mesocellular Carbon Foams with Uniform Ultralarge Mesopores . J. Am. Chem. Soc. , 123 : 5146 – 5147 .
- Kleitz , F. , Choi , S.H. and Ryoo , R. 2003 . Cubic Ia3d Large Mesoporous Silica: Synthesis and Replication to Platinum Nanowires, Carbon Nanorods and Carbon Nanotubes . Chem. Commun. , 17 : 2136 – 2137 .
- Liu , X. , Tian , B. , Yu , C. , Gao , F. , Xie , S. , Tu , B. , Che , R. , Peng , L. and Zhao , D. 2002 . Room-Temperature Synthesis in Acidic Media of Large-Pore Three-Dimensional Bicontinuous Mesoporous Silica with Ia3d Symmetry . Angew. Chem. Int. Ed. , 41 : 3876 – 3878 .
- Su , F.S. , Lu , C.Y. , Kuo , S.C. and Zeng , W.T. 2010 . Adsorption of CO2 on Amine-Functionalized y-Type Zeolites . Energy Fuels , 24 : 1441 – 1448 .
- Franchi , R.S. , Harlick , P.J.E. and Sayari , A. 2005 . Applications of Pore-Expanded Mesoporous Silica: 2. Development of a High-Capacity, Water-Tolerant Adsorbent for CO2 . Ind. Eng. Chem. Res. , 44 : 8007 – 8013 .
- Yue , M.B. , Sun , L.B. , Cao , Y. , Wang , Y. , Wang , Z.J. and Zhu , J.H. 2008 . Efficient CO2 Capturer Derived from as-Synthesized MCM-41 Modified with Amine . Chem. Eur. J. , 14 : 3442 – 3451 .
- Liu , Y. , Shi , J.J. , Chen , J. , Ye , Q. , Pan , H. , Shao , Z.H. and Shi , Y. 2010 . Dynamic Performance of CO2 Adsorption with Tetraethylenepentamine- Loaded KIT-6 . Micropor. Mesopor. Mater. , 134 : 16 – 21 .