Abstract
Mpemba paradox results from hydrogen-bond anomalous relaxation. Heating stretches the O:H nonbond and shortens the H‒O bond via Coulomb coupling; cooling reverses this process to emit heat at a rate depending on its initial storage. Skin ultra-low mass density raises the thermal diffusivity and favors outward heat flow from the liquid.
As the source and central part of all lives, hydrogen bond (O:H‒O, the ‘:’ is the lone pair and the ‘-’ bonding pair of electrons) plays key role in DNA and protein folding, drug-target binding, ion-channel activating/deactivating, gene delivering, cancel cell curing, messaging, and signaling, etc. However, response of hydrogen bond to temperature and coordination environment is crucial to these activities though mechanisms remain yet unknown. This article shows how the O:H‒O bond relaxes in resolving the Mpemba paradox based on our extensive research.Citation1
illustrates (a) the potential paths and (b) the initial temperature dependence of the liner velocity of the O:H‒O bond during thermal relaxation.Citation2 The hydrogen bond consists of the intermolecular O:H nonbond (left-hand side of the H+ reference origin) and the intramolecular H‒O bond (right-hand side) other than either of them alone. The O:H‒O bond acts as an asymmetric, H+ bridged, and coupled oscillator pair with memory, whose cooperative relaxation stems anomalies of water ice such as ice floating, regelation, ice slippery, hydrophobic and tough water skin, Hofmeister effect, etc.Citation1 An interplay of the O:H nonbond (van der Waals-like) interaction, the H‒O covalent bond interaction, the O‒O Coulomb repulsion and externally applied stimulus always dislocate O atoms in the same direction but by different amounts along the respective potential paths.Citation3 The softer O:H nonbond relaxes more than the stiffer H‒O does.
Figure 1. (A) O:H‒O bond asymmetric, short-range potential paths, being analogous to springs and (B) O:H‒O bond memory effect - initial temperature dependence of the H‒O bond relaxation velocity at cooling. Numerical duplicates the measured (insets) initial temperature dependence of the (C) θ(θi, t) curvesCitation7 and (D) the skin-bulk temperature difference θ(θi, t) of water at cooling.Citation2 The dH0 and dL0 in (A) are the respective references at 4°C. Potentials include the O:H nonbond interaction (vdW of EL ∼0.1 eV bond energy; left-hand side), the H‒O bond exchange interaction (EH ∼ 4.0 eV; right-hand side), and the Coulomb repulsion between electron pairs (paired green dots) on adjacent oxygen atoms. These interactions dislocate O atoms in the same direction by different amounts with respect to the H+ origin under excitation. The relaxation proceeds along the O:H‒O bond potentials from hotter (red line linked spheres, labeled ‘hot’) to colder state (blue line linked spheres, labeled ‘cold’).
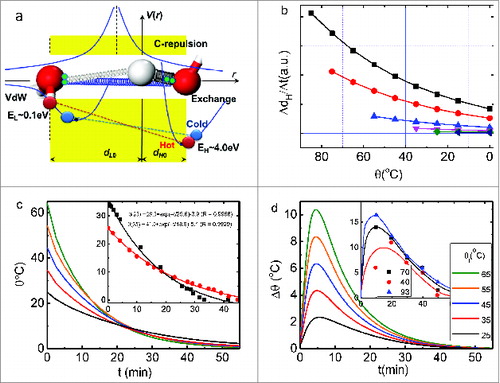
Generally, heating stores energy in a substance by stretching and softening all bonds involved. However, heating stores energy in water by stretching the O:H nonbond and simultaneously compressing the H‒O bond (red line linked spheres in are in the hot state) by the joint interactions. Cooling reverses (blue line linked spheres) this process, analogous to suddenly releasing a coupled, highly deformed bungee pair, one of which is stretched and the other compressed. This process emits energy at a rate that depends on the deformation history of the bungee pair (i.e., how much they were stretched or compressed).Citation4
The O:H‒O bond exhibits memory with thermal momentum during cooling. The linear velocity of the H‒O bond ΔdH/Δt in is the product of slopes for the known temperature dependence of H‒O length dH(θ) and the measured initial temperature and time dependence of water temperature θ(θi, t) shown in as inset. Because of the Coulomb coupling of the O:H and H‒O, the velocity Δdx/Δt and energy rate ΔEx/Δt (x = L for O:H and x = H for H‒O) can be readily derived but here we show the representative only for simplicity. indicates that the initially shorter H‒O bond at higher temperature remains highly active compared to its behavior otherwise when they meet on the way to freezing.Citation4
Conversely, molecular undercoordination (with fewer than 4 neighbor as in the bulk) has the same effect of heating on O:H‒O bond relaxation.Citation5 H‒O bond contracts and O:H expands, which shrinks molecular size and expands their separations with an association of polarization.Citation6 Water skin forms such a supersolid phase that is elastic, hydrophobic, ice like, and less dense (0.75 gcm−3). The lower mass density raises the thermal diffusivity and favors outward heat flow from the liquid.
show the theoretical duplication of measured (insets) Mpemba paradox.Citation4 The Mpemba paradox is characterized byCitation7: (i) hot water freezes faster than cold water under the same conditions; (ii) the liquid temperature θ drops exponentially with cooling time (t); (iii) water skin is warmer than sites inside the liquid and the skin of hotter water is even warmer.
Besides the thermal diffusivity and convection velocity involved in the non-linear Fourier thermal fluid transport equation, systematic examination of all possible boundary conditions of a 10-mm long, one-dimensional tube cell of water with a one-millimeter thick skin cooling from θi to θf = 0 °C revealed the following:
Characterized by the crossing temperature in , the Mpemba effect happens only in the presence of the supersolid skin.
The Mpemba effect is sensitive to the source volume, the extent of skin supersolidity, skin radiation and the drain temperature θf.
The thermal convection has little effect on observations.
It is necessary to emphasize that the Mpemba effect happens only in circumstances where the water temperature drops sharply from θi to θf at the source–drain interface. Larger liquid volume may prevent this effect by heat-dissipation hindering. Any spatial temperature decay between the source and the drain could prevent the Mpemba effect. Conducting experiments under identical conditions is necessary to minimize artifacts such as radiation, source/drain volume ratio, exposure area, container material, etc. Therefore, conditions for the Mpemba effect are indeed very critical, which explains why it is not frequently observed.
Therefore, O:H‒O bond memory and water-skin supersolidity determines the heat ‘emission-conduction-dissipation’ dynamics of the Mpemba paradox in the ‘source-path-drain’ cycle system and the skin-drain interface must be highly nonadiabatic.
Disclosure of Potential Conflicts of Interest
No potential conflicts of interest were disclosed.
References
- Huang Y, et al. Coord Chem Rev 2014 doi:http://dx.doi.org/10.1016/j.ccr.2014.10.003
- Sun C Q, et al. J Phy Chem Lett, 2013; 4:3238-3244
- Huang Y, et al. J Phys Chem B 2013; 117(43):13639-45; PMID:24090472; http://dx.doi.org/10.1021/jp407836n
- Zhang X, et al. Phys Chem Chem Phys, 2014; 16:22995-3002; PMID:25253165; http://dx.doi.org/10.1039/C4CP03669G
- Zhang X, et al. Phys Chem Chem Phys 2014; 16:22987-94; PMID:25198167; http://dx.doi.org/10.1039/C1034CP02516D
- Sun C Q, et al. J Phys Chem.Lett, 2013; 4:2565-2570.
- N Bregović. Mpemba effect from a viewpoint of an experimental physical chemist. http://www.rsc.org/images/nikola-bregovic-entry_tcm18-225169.pdf, 2012.