Abstract
The metal vibrating probe developed in the 1970s to measure electric current is sensitive down to the micro-Amp range but detects only net current due to flow of multiple ions, and is too large to measure from single cells. Electrophysiological techniques which use glass microelectrodes such as voltage clamping can be used on single cells but are also non-specific. Ion-selective probes are glass microelectrodes containing at their tip a small amount of ionophore permeable to a particular ion. The electrode is therefore sensitive to changes in concentration of this ion. If the probe tip is moved at low frequency between two points in a concentration gradient of this ion then the electrochemical potential of the solution inside the electrode fluctuates in proportion to the size of the ion gradient. This fluctuation is amplified and recorded and is used to calculate the actual ion flux using Fick’s law of diffusion. In this mini-review we describe the technique of ion-selective self-referencing microelectrodes to measure specific ion fluxes. We discuss the development of the technique and describe in detail the methodology and present some representative results.
Cells generate and maintain a significant electrical membrane potential by active transport of ions, mainly via the sodium-potassium exchange pump, which transports Na+ ions out of the cell and K+ ions in. This membrane potential allows the cell to function as a battery, powering a number of membrane processes including transport of sugars and other ions. The membrane potential of the mitochondria drives the production of ATP, the cell's energy source. Excitable cells such as neurons have large membrane potentials. Rapid collapse of this potential by opening of sodium channels and in-rush of Na+ ions (depolarization) produces the action potential which is the basis of nerve transmission and brain function. Many tissues and organs also have long-lasting electrical potentials. Epithelia (skin, cornea, etc.) generate and maintain trans-epithelial potentials by directional pumping of ions (Na+ and Cl−). Many years ago it was discovered that wounds on human skin generate significant electrical potentials.Citation1,Citation2 The development of the vibrating probe allowed these small bio-electric currents generated by cells and tissues to be measured non-invasively.Citation3,Citation4 This ultra-sensitive probe can measure extracellular currents in the micro-Amp (µA) range, but gives no indication of the ion species involved.
To measure specific ion flux one could use two or more ion-selective electrodes in different positions to detect the concentration gradient. However; the inherent voltage drift of the probes would be different, causing a change in signal even if the ion flux was stable. The solution was to use self-referencing whereby a single ion-selective probe is moved at low frequency between two points close together. Now, even if the electrode potential drifts, the flux measurement is stable because the drift is relatively slow so that the probe moves before significant drift can occur (see ). A low frequency vibrating ion-selective electrode was first developed in 1990 to measure specific calcium flux.Citation5 The tip of the glass micro-electrode contains an ionophore permeable to only Ca2+ ions, so the electrode is sensitive to changes in [Ca2+]. As the electrode moves back and forth in a gradient (flux) of Ca2+, pausing at each position, the electrochemical voltage of the electrode changes in proportion to the size of the ion flux. The electrode signal is amplified and recorded to computer. The ion flux can be calculated using Fick's law of diffusion: J = Cu(dc/dx) where C is the ion concentration in the solution; u is the ion mobility; and dc is the concentration difference over distance dx. The electrode potential in standard solutions containing different ion concentrations is used to construct a calibration curve to calculate the actual ion concentration. As well as Ca2+, commercially available ionophores are now available to make electrodes sensitive to Na+, Cl−, K+, H+, Mg2+, nitrate, ammonium, fluoride, lithium, mercury, etc. (see www.sigmaaldrich.com).
Ion-selective probes have been used to measure H+, K+ and Ca2+ flux across plant roots,Citation6,Citation7 Cl− flux in rat cerebral arteries,Citation8 Cl− flux in pollen tubes,Citation9 H+ flux in skate retinal cells,Citation10 Ca2+ flux in mouse bone,Citation11 various ion fluxes in fungal hyphae,Citation12,Citation13 and various ion fluxes in rat cornea.Citation14 See also the following reviews for detailed information on self-referencing ion-selective electrodes. Citation15–Citation17 Interesting recent developments include amperometric self-referencing detectors of oxygen, nitric oxide and neurotransmitters dopamine and glutamate.Citation18,Citation19 Amperometric sensing is based on a chemical reaction at the sensor tip. New fiber-optic microprobes (optrodes) have been developed to measure non-invasively oxygen metabolic flux with high selectivity and sensitivity. Citation20,Citation21 There is now also an enzyme-based nanoparticle-coated probe sensitive to glucose.Citation22
Ion-selective microelectrodes are best made with thin-walled glass capillaries such as World Precision Instruments (WPI) borosilicate glass capillaries without filament (10 cm long, 1.5 mm outer diameter, 1.12 mm inner diameter, cat # TW150-4). Electrodes are heat-pulled using a Sutter P-97 electrode puller with the following settings: heat 470, pull 13, velocity 15, delay 1. This gives tips 3–4 µm in diameter. Smaller tips have higher resistance which makes them more susceptible to electronic noise. The electrodes are now dried and rendered hydrophobic by silanization. Electrodes are placed in a metal rack and heated overnight in an oven at 200°C. About 1–2 ml of silanization solution I (Sigma-Aldrich, cat # 85126) is placed in a glass Petri dish in the bottom of the oven. Electrodes are kept in the oven until all the silanization solution had vaporized; then the oven is turned off. After cooling, electrodes are stored in an electrode storage jar inside a glass dessicator. Electrodes can be stored thus for many weeks.
To prepare an ion-selective probe (); the electrode is first back-filled to a length of about 1 cm with a solution containing 100 mM of the ion to be measured (see ). This is done using a disposable plastic Pasteur pipette heat-pulled in a Bunsen burner to a fine filament. To eliminate the air bubble at the electrode tip, the electrode is attached to a 3 ml syringe via a silicon tube (3 mm o.d, 1.5 mm i.d). The electrode is secured with sticky-tack on the stage of a Nikon inverted microscope and observed at low power (x4 lens) while pressure is applied by the syringe to push the air bubble out the tip. The electrode is then tip-filled with a length of 30–50 µm of ion-specific ionophore (see ). A small droplet of ionophore is placed on the short edge of a microscope slide. The electrode tip is observed with a x10 lens and the microscope slide moved towards it until the electrode tip touches the ionophore. The ionophore is drawn into the electrode by capillary pressure. A long column of ionophore should be avoided as this increases the probe's electrical resistance which can make it susceptible to electroinic interference (noise). The electrode is mounted in a straight microelectrode holder with a gold 1 mm male connector and Ag/AgCl wire () (Warner Instruments, cat # QSW-A15P). The electrode holder is mounted on a Newport 3-dimensional computer-controlled electronic micro-positioner (see below). The electrode tip is place in measuring solution appropriate for the sample to be measured (physiological saline, culture medium, etc.) to allow the electrode to stabilize for an hour or two, or even overnight.
Reference electrodes () are the same glass capillaries as above; cut into 5 cm lengths and fire-polished at each end for 1–2 sec in a Bunsen flame. These electrodes are filled with a 3 M solution of NaCl, CH3CO2K (potassium acetate) or KCl, with 2% agar. The solution is chosen depending on the ion to be measured (the reference electrode cannot contain the ion being measured; see ). Agar (0.2 g) is added to 10 ml of solution and brought to the boil on a hotplate. The reference electrode is attached to a plastic Pasteur pipette and the hot solution drawn into the capillary. The electrode is then dropped into cold 3 M NaCl, CH3CO2K or KCl solution and stored in 3 M solution in Petri dishes prior to use. Reference electrode with air bubbles are discarded. The reference electrode is mounted in a straight microelectrode holder (pre-filled with 3 M solution) with a Ag/AgCl pellet inside and a gold 2 mm male connector () (WPI, cat # MEH3S) and mounted on a Newport 3-dimensional micro-positioner.
Before experiments, consideration must be taken of the sample to be measured, and how the sample is to be immobilized and mounted for measurements. For example, for cornea measurements we glue two wire loops into a 9 cm or 5 cm plastic Petri dish (). The loops hold the eye stable but allow it to be rotated or tilted to give the electrode access to different parts of the cornea.Citation14 For tadpole measurements we glue a plastic ‘shelf’ and fine wire into a 5 cm Petri dish (). The loop in the wire holds the tadpole stable without damage.
Electrode movement and data recording are controlled by IonView32 software (BioCurrents Research Center; Marine Biological Laboratory, Woods Hole, MA). The ion-selective electrode moves at low frequency (0.3 Hz) between two points 30 µm apart (). The electrode pauses at each position and the electrode potential in mV is recorded on the computer (). If an ion flux is present, the electrode detects a difference in ion concentration between the two positions. The actual ion flux is calculated using Fick's law of diffusion: J = Cu(dc/dx) where C is the ion concentration in the solution, u is the ion mobility, and dc is the concentration difference over distance dx. Ion flux data are presented in pmol/cm2/sec or nmol/cm2/sec.
Before and after experiments, electrodes are calibrated in three standard solutions. These solutions should contain ion concentrations above and below the ion concentration in the measuring solution. For example, for Na+ cornea measurements in artificial tear solution (BSS+ Intraocular Irrigating Solution, Alcon Laboratories, Inc.,) which contains ∼150 mM Na+, calibrating solutions contain 10, 100 and 200 mM NaCl. The Ca2+ concentration is much lower so a Ca2+ electrode is calibrated in 0.1, 1 and 10 mM CaCl2.2H2O. Plotting the electrode output (mV) against the logarithm of the molar ion concentration usually gives a linear correlation with an R2 value close to 1 (). The formula describing the line is used to convert the raw output of the electrode in mV into actual ion concentrations, and in turn the ion flux is calculated using the formula above.
We measured Ca2+ and K+ fluxes at a cornea wound over time (, data adapted from Vieira et al. 2011).Citation14 The data are normalized because the K+ flux is much larger than the Ca2+ flux. Before wounding (time zero) cornea has small outward flux of both ions (efflux). After wounding, K+ flux increases dramatically but then drops back down after 20 min. This suggests that the K+ flux is leakage from damaged cells, which have a high intracellular [K+]. We confirmed this using a high external K+ concentration. In high [K+] the initial peak of K+ flux was absent.Citation14 In contrast to K+ flux, Ca2+ flux increased slowly and was maintained at a significantly higher level. This suggests that Ca2+ efflux is an active response to cornea wounding. Chemical fixation of the cornea eliminated the Ca2+ flux.Citation14
In conclusion, ion-selective self-referencing probes are extremely useful tools where small fluxes of specific ions need to be measured from tissues or even single cells. They have proved useful in a wide variety of biological applications.Citation6–Citation14 New adaptations of self-referencing include the use of amperometric,Citation18,Citation19 opticalCitation20,Citation21 and nanoparticle-coated sensors.Citation22
Figures and Tables
Figure 1 Ion-selective electrodes. A) NA+-selective electrode (left) and reference electrode (right). B) Ion-selective (left) and reference (right) electrodes mounted in their respective holders. C) Custom-made dishes for mounting and measuring from eyes (left) and tadpoles (right).
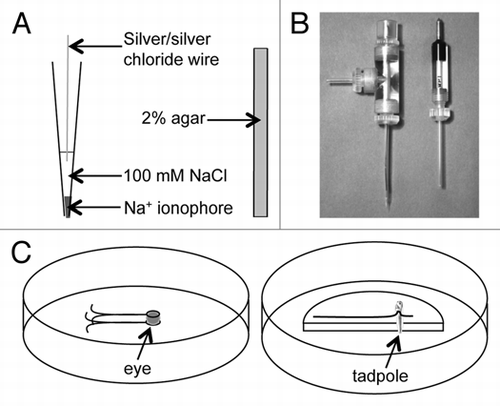
Figure 2 Measuring and calibration. (A) As the ion-selective electrode moves in an ion gradient, near a source or sink of ion flux, the potential on the electrode varies in proportion to the size of the flux (B). The higher value at the near position indicates an outward ion flux (efflux) in this case. This is an actual trace recorded from a Ca2+ electrode near a corneal wound. note that although there is some slight drift downwards in the trace the actual flux (difference between near and far) is quite consistent. Time between points on the x-axis is 6 sec. (C) Calibration of the Ca2+ electrode used in (B) in three solutions (0.1, 1 and 10 mM CaCl2.2H2O; logs of molar concentration: -4, -3, -2). The formula describing the linear best fit line is used to calculate the actual ion flux (see Methods).
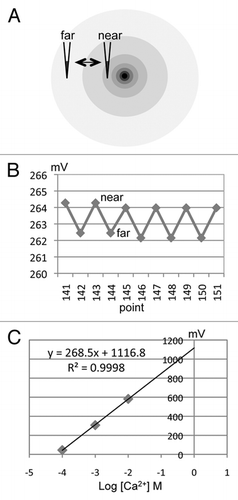
Figure 3 Cornea wound measurements. Cornea wounding induces different K+ and Ca2+ fluxes. After wounding (at time zero), K+ flux rises and falls rapidly, suggesting this is passive leakage from damaged cells, which contain high [K+]. In contrast, Ca2+ flux rises slowly and is maintained at a high level, suggesting that Ca2+ efflux is an active response to corneal injury. Data adapted from Vieira et al., 2011.Citation14
![Figure 3 Cornea wound measurements. Cornea wounding induces different K+ and Ca2+ fluxes. After wounding (at time zero), K+ flux rises and falls rapidly, suggesting this is passive leakage from damaged cells, which contain high [K+]. In contrast, Ca2+ flux rises slowly and is maintained at a high level, suggesting that Ca2+ efflux is an active response to corneal injury. Data adapted from Vieira et al., 2011.Citation14](/cms/asset/ff1ae060-2063-403f-9719-25b1466c2d8b/kcib_a_10916182_f0003.gif)
Table 1 Examples of commonly used ionophores
Acknowledgments
This work was supported by the National Institutes of Health National Eye Institute grant 1R01EY019101 (to M.Z. and B.R.). The authors thank the Wellcome Trust for continuous support (068012). This work was also supported in part by Research to Prevent Blindness, Inc., an NSFC grant (30628026), and UC Davis Dermatology Department developmental fund. M.Z. is also supported by grants from the California Institute of Regenerative Medicine RB1-01417, NSF MCB-0951199.
References
- Du Bois-Reymond E. Vorläufiger abriss einer untersuchung uber den sogenannten froschstrom und die electomotorischen fische. Ann Phy U Chem 1843; 58:1 - 30
- Piccolino M. Animal electricity and the birth of electrophysiology: the legacy of Luigi Galvani. Brain Res Bull 1998; 46:381 - 407
- Jaffe LF, Nuccitelli R. An ultrasensitive vibrating probe for measuring steady extracellular currents. J Cell Biol 1974; 63:614 - 628
- Reid B, Nuccitelli R, Zhao M. Non-invasive measurement of bioelectric currents with a vibrating probe. Nat Protoc 2007; 2:661 - 669
- Kühtreiber WM, Jaffe LF. Detection of extracellular calcium gradients with a calcium-specific vibrating electrode. J Cell Biol 1990; 110:1565 - 1573
- Ryan PR, Newman IA, Shields B. Ion fluxes in corn roots measured by microelectrodes with ion-specific liquid membranes. J Memb Sci 1990; 53:59 - 69
- Kochian LV, Shaft JE, Kühtreiber WM, Jaffe LF, Lucas WJ. Use of an extracellular; ion-selective; vibrating microelectrode system for the quantification of K+, H+ and Ca2+ fluxes in maize roots and maize suspension cells. Planta 1992; 188:601 - 610
- Doughty JM, Langton PD. Measurement of chloride flux associated with the myogenic response in rat cerebral arteries. J Physiol 2001; 534.3:753 - 761
- Messerli MA, Smith PJS, Lewis RC, Robinson KR. Chloride fluxes in lily pollen tubes: a critical reevaluation. Plant J 2004; 40:799 - 812
- Molina AJ, Verzi MP, Birnbaum AD, Yamoah EN, Hammar K, Smith PJ, Malchow RP. Neurotransmitter modulation of extracellular H+ fluxes from isolated retinal horizontal cells of the skate. J Physiol 2004; 560:639 - 657
- Marenzanaa M, Shipley AM, Squitierob P, Kunkelb JG, Rubinaccia A. Bone as an ion exchange organ: Evidence for instantaneous cell-dependent calcium efflux from bone not due to resorption. Bone 2005; 37:545 - 554
- Ramos AC, Façanha AR, Feijó JA. Proton (H+) flux signature for the presymbiotic development of the arbuscular mycorrhizal fungi. New Phytol 2007; 178:177 - 188
- Lew RR. Ionic currents and ion fluxes in Neurospora crassa hyphae. J Exp Bot 2007; 58:3475 - 3481
- Vieira AC, Reid B, Cao L, Mannis MJ, Schwab IR, Zhao M. Ionic components of electric current at rat corneal wounds. PLoS One 2011; 6:17411
- Smith PJS. Non-invasive ion probes—tools for measuring transmembrane ion flux. Nature 1995; 378:645 - 646
- Smith PJS, Hammar K, Porterfield DM, Sanger RH, Trimarchi JR. Self-referencing, non-invasive, ion selective electrode for single cell detection of trans-plasma membrane calcium flux. Microsc Res Technique 1999; 46:398 - 417
- Smith PJS, Sanger RH, Messerli MA. Michael AC, Borland LM. Principles, development and applications of self-referencing electrochemical microelectrodes to the determination of fluxes at cell membranes. Electrochemical Methods for Neuroscience 2007; Boca Raton CRC Press 18
- Porterfield DM. Measuring metabolism and biophysical flux in the tissue, cellular and sub-cellular domains: recent developments in self-referencing amperometry for physiological sensing. Biosens Bioelectron 2007; 22:1186 - 1196
- McLamore ES, Mohanty S, Shi J, Claussen J, Jedlicka SS, Rickus JL, Porterfield DM. A self-referencing glutamate biosensor for measuring real time neuronal glutamate flux. J Neurosci Methods 2010; 189:14 - 22
- Chatni MR, Porterfield DM. Self-referencing optrode technology for non-invasive real-time measurement of biophysical flux and physiological sensing. Analyst 2009; 134:2224 - 2232
- McLamore ES, Jaroch D, Chatni MR, Porterfield DM. Self-referencing optrodes for measuring spatially resolved, real-time metabolic oxygen flux in plant systems. Planta 2010; 232:1087 - 1099
- McLamore ES, Shi J, Jaroch D, Claussen JC, Uchida A, Jiang Y, et al. A self referencing platinum nanoparticle decorated enzyme-based microbiosensor for real time measurement of physiological glucose transport. Biosens Bioelectron 2011; 26:2237 - 2245