Abstract
Mechanosensitive ion channels have long been the only established molecular class of cell mechanosensors with known molecular entities. However, recent advances in the state-of-the-art techniques, including single-molecule manipulation and imaging, have enabled an investigation of non-channel type cell mechanosensors and the underlying biophysical mechanisms of their activation. To date, two focal adhesion proteins, talin and p130Cas, have been postulated to act as putative mechanosensors, acting through mechano-induced unfolding of their particular soft domain(s) susceptible to phosphorylation. More recently, the actin filament has been demonstrated to act as a mechanosensor in the presence of the soluble actin-severing protein, cofilin. The cofilin severing activity negatively depends on the tension in the actin filament through tension-dependent binding/unbinding of cofilin to/from the actin filament. As a result, relaxed actin filaments are severed, while tensed ones are either not severed or severed after a long delay. Here we review the latest progress in the mechanosensing by non-channel type proteins and discuss the possible physiological roles of the mechanosensing performed by actin filaments in the course of cell migration.
Introduction: Stress concentration Sites and Mechanosensing in the Cell
The hundred trillions of cells which make up our bodies are continually exposed to various mechanical stimuli, including muscle contraction, ongoing blood flow, blood pressure, distension of visceral organs, etc., which initiate a wide range of cellular responses. These responses include Ca2+ mobilization,Citation1,Citation2 protein phosphorylation,Citation3-Citation5 rearrangement of the cytoskeleton,Citation6,Citation7 transcriptional regulation,Citation8 apoptotic cell death,Citation9,Citation10 and cell differentiationCitation11 and so on. Mechanical forces are sensed by mechanosensors that presumably undergo change in their enzymatic activity or interaction with signaling molecules in response to forces. However, the particular molecular entities and the underlying biophysical mechanisms of these mechanosensing molecules are largely unknown except for the mechanosensitive (MS) channels.Citation12-Citation14 A major reason for this slow progress is that mechanosensors, by their nature, do not possess the specific chemical ligands such as agonists, antagonists and inhibitors, which are used as biochemical tools to detect and purify receptor molecules. An alternative way toward the molecular identification of mechanosensors relies on an idea that putative mechanosensors are most likely localized at cellular sites of high concentrations of stress. The cell membrane is such a structure due to its high lateral elastic modulus, and is actually endowed with MS channels, although the correlation between the highly stressed membrane region and the MS channel localization has yet to be demonstrated. Here we focus on adhesive structures, including focal adhesions, the actin cytoskeleton, and the molecular apparatus connecting these structures, where stress is presumably highly concentrated.
Generally focal adhesions comprise a high stress concentration site, linking extracellular matrices and the actin cytoskeleton.Citation15 Mechanical forces imposed from inside or outside of the cell are transmitted through the focal adhesions bidirectionally, i.e., in an outside-in or inside-out direction.Citation16 Exogenous mechanical forces are exerted on integrins, an extracellular matrix receptor enriched in focal adhesions that activate a variety of intracellular signaling cascades.Citation17-Citation19 The activities of actin modulating proteinsCitation20 are also influenced by endogenous cell contractile forceCitation21 or exogenous mechanical stimuli.Citation6,Citation7 Thus, mechanosensorsCitation22 and directly associated signaling moleculesCitation15 are thought to be involved in the focal adhesion, the actin cytoskeleton and/or cellular structures linking focal adhesions and the actin cytoskeleton.
A recent in vitro biophysical study has shown that the apparent actin filament severing activity of cofilin and the rates of binding of cofilin to actin filaments are both affected by the tension present in the actin filaments,Citation23 implying that the actin filament itself works as a mechanosensor.
Here, we review the recent progress in the study of tension-sensing by focal adhesion proteins and actin filaments, and evaluate the possible physiological roles of such tension-sensing by actin filaments.
Tension Sensing by Focal Adhesion Proteins
Mounting evidence suggests that focal adhesion proteins are involved in the mechanically triggered activation of intracellular signaling molecules,Citation15 including MAP kinases,Citation24 Akt,Citation25,Citation26 and PI3 kinase.Citation27 Direct manipulation and imaging of single protein molecules enables an application of mechanical forces to a target protein while monitoring its response, using these methods talinCitation28 and possibly p130CasCitation29 have been proposed to work as a mechanosensor.
The focal adhesion protein talin has a head domain that binds to the cytoplasmic tail of the integrin β subunit, while its rod domain contains actin-binding sites, each of which locates adjacent to a vinculin-binding site. When mechanical force was applied to talin through the manipulation of a small bead attached on a focal adhesion, integrin-cytoskeleton linkage at the focal adhesion was strengthenedCitation30 and vinculin was translocated to the focal adhesion underneath the bead in vivo,Citation31 suggesting that talin acts as a mechanosensor in focal adhesions.Citation30,Citation32
A recent in vitro study demonstrated tension-sensing by the talin rod domain. The recombinant talin rod domain was extended approximately 100 nm by direct application of force (20 pN)Citation28 with an AFM tip (), resulting in an increase in the number of vinculin head domains bound to the talin rod domain. Combining molecular and cellular level studies, the authors have proposed that the force applied through the integrins in the focal adhesions extends the talin rod domain and exposes its binding sites for vinculin, which reinforces the actin-integrin linkage in vivo.
Figure 1. (A) Structure of the talin rod domain consisting of 12 helices (upper panel). The rod domain which is unfolded under forceCitation28 (lower panel) is shown by the black arrows in the upper panel. (B) Schematic drawing of the experimental setup to apply force to a single actin filament.Citation23 One end of an actin filament (red) was tethered to a bead fixed on a coverslip, and the other end of the filament was tethered to a small bead manipulated by optical tweezers. The lower actin filament is not tensed, and is severed by cofilin. (C) (i), Schematic drawing of the experimental setup to trace the torsional fluctuations of a single actin filament. An actin filament is tethered on the coverslip via gelsolin, and a bead was attached to the lower end of the actin filament. Rotation of the bead is monitored using fluorescent small beads that are attached on the large bead. (ii), Rotational angular fluctuations of a bead attached to an actin filament during the time the large bead was trapped, but not stretched, by optical tweezers. (iii). The rotational angular fluctuations were decreased when the actin filament was stretched by moving the trapping point in downward direction. The data show the results at zero and ca. 5 pN stretch force; one can change the applied force by increasing the laser power of the optical tweezers. Panels A and C are based on studies (28) and (23), respectively.
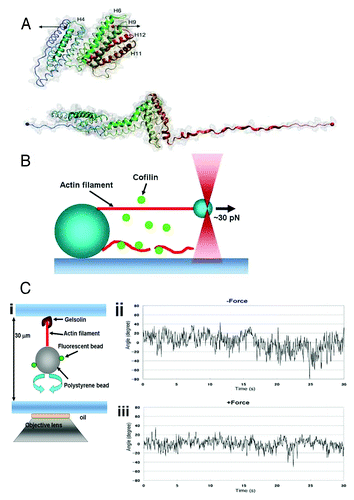
p130Cas, a substrate for p60Src,Citation29 is a scaffolding protein that localizes at focal adhesions. Stretching of the cell substrate induces tyrosine phosphorylation of p130Cas, followed by the activation of the p38 MAP kinase cascade via small GTPase Rap1Citation33 in intact cells, suggesting that p130Cas is involved in mechanically-induced signal transduction. A recent biophysical study using the purified p130cas substrate domain for p60Src suggested that p130Cas might work as a mechanosensor. Mechanical extension of the elastic substrate, to which the purified recombinant p130Cas substrate domain is adhered, induces tyrosine phosphorylation of the p130Cas substrate domain by p60Src, implying that the mechanical unfolding of the p130Cas substrate domain increases the phosphorylation level of the p130Cas not only in vitro but also in vivo.Citation34 However, further studies are needed to confirm this phenomenon actually takes place in live cells.
In intact spreading cells, the staining pattern made by an antibody against the extended p130Cas substrate domain shows a similar staining pattern to that made by an antibody against phosphorylated p130Cas in the cell periphery,Citation34 suggesting that the p130Cas substrate domain is extended by force, and tyrosine-phosphorylated by p60Src.Citation33 In these mechanosensing processes, the unfolding of the sensor molecules (talin and p130Cas) is presumably required to sense forces and to transmit them to the downstream signaling molecules.
Tension Sensing by Titin and Fibronectin
Titin, a giant elastic muscle protein connecting the Z-disc and M-line in the sarcomere, is unfolded by force in its kinase domain.Citation35 The improper unfolding of the kinase domain is thought to be involved in muscle disuse atrophy.Citation36 Fibronectin, an extracellular matrix protein, is mechanically unfolded by cell contractile forces,Citation37 and the unfolding may be involved in accelerated fibronectin assembly, resulting in an enhancement of the fibronectin-integrin linkage.Citation38,Citation39
Tension Sensing by Actin Filaments
A recent in vitro study revealed that the actin filament itself functions as a mechanosensor.Citation23 One end of a single actin filament was tethered to a myosin-coated bead fixed on a coverslip, while the other end was tethered to a small myosin coated bead manipulated with optical tweezers so as to tense the filament (). When the filament was tensed (~30 pN), it was severed by cofilin with a larger delay compared with the filament when it was not tensed, or was not severed within the observation period (ca. 30 sec). Additionally, the binding of cofilin to the bundles of actin filaments was imaged with fluorescein labeled cofilin, which showed that the rate of the binding of cofilin to the actin bundles decreased when the bundles were tensed. Approximately 2 pN of force is sufficient to decrease the apparent severing activity of cofilin,Citation23 which is comparable to the force generated by a single myosin head. A single actin stress fiber is composed of 10–30 actin filaments,Citation40 and the contractile force in a single stress fiber is estimated to be on the order of nN,Citation41 suggesting that the contractile force in stress fibers (> 2 pN for each actin filament, assuming the stress in the stress fiber is evenly distributed among the actin filaments) is high enough to prevent cofilin from binding to the actin filaments in vivo.
How does tension prevent the binding of cofilin to the actin filaments? There are enormous numbers of biochemical,Citation42,Citation43 structural,Citation44,Citation45 and computationalCitation46 studies on the binding of cofilin to actin filaments. Electron microscopic analyses revealed that the twist of the actin filament is increased when the filament is fully decorated by cofilin.Citation44,Citation45 On the other hand, the rotational angular conformation of actin protomers in native actin filaments is variableCitation47; the angle between neighboring actin protomers reportedly ranges from 156° to 170°.Citation45 Based on these observations, it is hypothesized that cofilin preferentially binds to actin filaments in solution when the protomers of the actin filament are in the twisted state; i.e., large torsional fluctuations are required for the binding of cofilin to the filament.
The torsional fluctuations of single actin filaments were visualized by monitoring the rotation of a bead attached on one end of the filament (). Application of a force of approximately 5 pN reduced the torsional fluctuations of the filament (), indicating that the actin filament fluctuates less when the filaments are tensed, supporting the hypothesis that tension in the actin filament reduces torsional fluctuations of the actin filament, which decreases the effective number of cofilin binding sites so as to prevent the binding of cofilin, resulting in an inhibition of the cofilin severing of the filament. This may constitute the potential tension sensing mechanism performed by actin filaments. Here, the sensor molecule actin filament senses the applied force and transduces it into changes in the fiber fluctuation that in turn modulates the activity (binding here) of the signaling molecule cofilin, eventually regulating the fiber dynamics by itself. This forms a kind of very smart and reliable local feedback regulatory system.
Possible Roles of Tension Sensing by Actin Filaments in Cells Responding to Mechanical Forces
The tension-dependent local disassembly of actin filaments by cofilin presumably works under certain specific physiological conditions. The distribution of cofilin in living cells was examined using a GFP-cofilin fusion protein. GFP-cofilin translocated to the stress fibers within a period of one minute when tension in the stress fibers was reduced by relaxing the pre-stretched elastic cell substratum (), followed by disassembly of the stress fibers,Citation23 suggesting that cofilin mediates disassembly of the stress fibers with a decline in tension in living cells. BDM, a myosin ATPase inhibitor, reduces tension in the stress fibers, and induces stress fiber disassembly in living cells. Stress fiber disassembly by BDM is reportedly enhanced in cells overexpressing cofilin.Citation21 By contrast, stress fiber disassembly was not detected in the cells without BDM, strongly supporting the proposal that cells make use of the tension-dependent local disassembly of actin filaments by cofilin.
Figure 2. (A) When a certain amount of tension was generated in the stress fibers in adherent cells, the binding of cofilin to the stress fibers was reduced (upper panel). When the tension was reduced by relaxing the cell substratum (the direction is indicated by the black arrows), cofilin bound and started to disassemble the stress fibers (lower panel). (B) Schematic drawing of the actin cytoskeleton in a locomoting keratocyte. The actin filaments are disassembled by cofilin near the leading edge of the cell. The prominent transverse stress fibers generating a large amount of contractile force are not disassembled. (C) Schematic drawing of the actin cytoskeleton in an adherent cell during migration. The actin filaments are disassembled in the trailing region of the cell, where the tension in the stress fibers is low, while the stress fibers generating tension in the middle region of the cell are not disassembled. The open arrows in B and C denote the direction of cell migration. The double-headed arrows indicate the width of the lamellipodia.
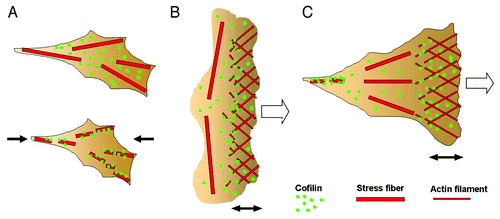
In accord with the above hypothesis, a tension decline in the actin stress fibers leads to the disassembly of the stress fibers, however, the possibility cannot be excluded that it is the disassembly that leads to the decline in tension in the stress fibers. To resolve this problem, simultaneous measurement of the tension decline and the disassembly of the actin stress fibers is required. We recently examined the relationship between the tension decline and stress fiber disassembly in living cells.Citation48 Fibronectin-conjugated beads were adhered to the endothelial cells in that study. Actin stress fibers were formed between focal adhesions underneath the bead and the focal adhesions at the cell bottom. Mechanical force was locally applied to the stress fibers by displacing the bead. The force (35 nN generated by 1 μm displacement of the bead) applied to the stress fibers and to the connected focal adhesions was estimated from the displacement of the beads embedded in the elastic substrate of the cells. When the attached bead was displaced, the force applied to the focal adhesions transiently rose, and then declined in less than a few seconds, indicating a tension decline in the stress fibers, probably due to a partial destruction of the linkage among the actin fibers within the stress fiber, which would decrease its elastic modulus. Subsequently, the same stress fibers were gradually disassembled within a period of 10 min, indicating that the decrease in tension in a particular stress fiber is followed by its disassembly, though the involvement of cofilin with this arrangement has not been examined yet.
Involvement of Tension Sensing by Actin Filaments in Migrating Cells
The potential roles of the tension-dependent local disassembly of actin stress fibers in migrating cells can be discussed in cells such as keratocyte and osteocarcinoma cells, in which the magnitude and distribution of the intrinsic contractile force generated by the actin cytoskeletons were quantitatively analyzed.Citation49-Citation51 The traction forces in migrating keratocytes were estimated from the local distortion in the elastic substratum of the cells. The traction forces generated beneath the front area of a migrating keratinocyte cell are relatively small, while the forces beneath the lateral area of the cell are substantial.Citation49 In locomotive keratocytes, actin filaments are polymerized near the leading edge of the cells and then disassembled within a few minutes, thereby maintaining a filament length of a few μm, while, in contrast, the prominent transverse stress fibers that connect both the lateral sides of the cell are not disassembled.Citation50 Similar prominent stress fibers connecting the lateral sides of the cells are also found in migrating osteosarcoma cells, and are disassembled with a decline in tension by the myosin inhibitor, blebbistatin.Citation51 The width of lamellipodia (shown by the double-headed arrow in ) is almost proportional to the length of the actin filaments extending at the leading edge, and knockdown of cofilin by RNAi increases the width of lamellipodia,Citation52 suggesting that cofilin mediates the actin filament disassembly near the leading edge. These findings fit the hypothesis that the disassembly of stress fibers by cofilin is inhibited when the filaments are tensed.
The tension across vinculin, a focal adhesion protein, has been assessed in the migrating endothelial cells using a vinculin-fusion protein named “a tension sensor module”Citation53 that was designed based on the FRET mechanism. The FRET signal is high at disassembling or sliding focal adhesions near the trailing edge of migrating cells, implying that the tension sensed by the sensor is low (< 2.5 pN) where stress fibers are disassembled. This also agrees with the above hypothesis, and suggests that a force as small as in a pN range can presumably be sensed by actin filaments, and, when decreased, initiates the stress fiber disassembly in living cells. Proper disassembly of stress fibers is crucial for cell migration, because actin stabilization by phalloidin,Citation54 and enhancement of stress fiber formationCitation55 both inhibit cell migration. Thus, the selective disassembly of non-tensed stress fibers is crucial for cell migration; e.g., cell migration is partly realized by the continual processing of actin fiber dynamics, including disassembly at the trailing edge and assembly at the leading edge of cells.
The invasion of cancer cells is enhanced by mechanical stimulation; the number of cancer cells that invaded the three dimensional matrix gel was increased when the gel was deformed by twisting small magnet beads embedded in the gel by an application of an external magnet field (i.e., mechanical stimulation to the cells in the gel). In addition, cofilin is involved in the mechanically stimulated invasion,Citation56 suggesting that the mechanosensing by actin filaments plays an important role in cancer cell invasion. Elucidating the role of the tension sensing performed by actin filaments in combination with cofilin binding/unbinding will ultimately provide great insights into cell behaviors under not only physiological, but also pathophysiological conditions.
Acknowledgments
This work was supported by a Grant-in-Aid from the Ministry of Education, Culture, Sports, Science, and Technology, Japan (to H.T. and M.S.), a grant from the Japan Space Forum (to H.T. and M.S.), NeuroCreative Lab (to HT), and the Japan Society for the Promotion of Science (JSPS) through its “Funding Program for World-Leading Innovative R&D on Science and Technology.”
References
- Naruse K, Sokabe M. Involvement of stretch-activated ion channels in Ca2+ mobilization to mechanical stretch in endothelial cells. Am J Physiol 1993; 264:C1037 - 44; PMID: 8386448
- Ando J, Komatsuda T, Kamiya A. Cytoplasmic calcium response to fluid shear stress in cultured vascular endothelial cells. In Vitro Cell Dev Biol 1988; 24:871 - 7; http://dx.doi.org/10.1007/BF02623896; PMID: 3170444
- Osawa M, Masuda M, Kusano K, Fujiwara K. Evidence for a role of platelet endothelial cell adhesion molecule-1 in endothelial cell mechanosignal transduction: is it a mechanoresponsive molecule?. J Cell Biol 2002; 158:773 - 85; http://dx.doi.org/10.1083/jcb.200205049; PMID: 12177047
- Liu M, Qin Y, Liu J, Tanswell AK, Post M. Mechanical strain induces pp60src activation and translocation to cytoskeleton in fetal rat lung cells. J Biol Chem 1996; 271:7066 - 71; http://dx.doi.org/10.1074/jbc.271.12.7066; PMID: 8636139
- Sai X, Naruse K, Sokabe M. Activation of pp60(src) is critical for stretch-induced orienting response in fibroblasts. J Cell Sci 1999; 112:1365 - 73; PMID: 10194415
- Shirinsky VP, Antonov AS, Birukov KG, Sobolevsky AV, Romanov YA, Kabaeva NV, et al. Mechano-chemical control of human endothelium orientation and size. J Cell Biol 1989; 109:331 - 9; http://dx.doi.org/10.1083/jcb.109.1.331; PMID: 2545727
- Hayakawa K, Sato N, Obinata T. Dynamic reorientation of cultured cells and stress fibers under mechanical stress from periodic stretching. Exp Cell Res 2001; 268:104 - 14; http://dx.doi.org/10.1006/excr.2001.5270; PMID: 11461123
- Yamazaki T, Komuro I, Yazaki Y. Molecular mechanism of cardiac cellular hypertrophy by mechanical stress. J Mol Cell Cardiol 1995; 27:133 - 40; doi.org/10.1016/S0022-2828(08)80013-2 http://dx.doi.org/10.1016/S0022-2828(08)80013-2; PMID: 7760338
- Grinnell F, Zhu M, Carlson MA, Abrams JM. Release of mechanical tension triggers apoptosis of human fibroblasts in a model of regressing granulation tissue. Exp Cell Res 1999; 248:608 - 19; doi.org/10.1006/excr.1999.4440 http://dx.doi.org/10.1006/excr.1999.4440; PMID: 10222153
- Chiu WT, Wang YH, Tang MJ, Shen MR. Soft substrate induces apoptosis by the disturbance of Ca2+ homeostasis in renal epithelial LLC-PK1 cells. J Cell Physiol 2007; 212:401 - 10; doi.org/10.1002/jcp.21037 http://dx.doi.org/10.1002/jcp.21037; PMID: 17311296
- Engler AJ, Sen S, Sweeney HL, Discher DE. Matrix elasticity directs stem cell lineage specification. Cell 2006; 126:677 - 89; http://dx.doi.org/10.1016/j.cell.2006.06.044; PMID: 16923388
- Sachs F. Mechanical transduction by membrane ion channels: a mini review. Mol Cell Biochem 1991; 104:57 - 60; http://dx.doi.org/10.1007/BF00229804; PMID: 1717821
- Sukharev SI, Blount P, Martinac B, Kung C. Mechanosensitive channels of Escherichia coli: the MscL gene, protein, and activities. Annu Rev Physiol 1997; 59:633 - 57; http://dx.doi.org/10.1146/annurev.physiol.59.1.633; PMID: 9074781
- Gillespie PG, Walker RG. Molecular basis of mechanosensory transduction. Nature 2001; 413:194 - 202; http://dx.doi.org/10.1038/35093011; PMID: 11557988
- Geiger B, Bershadsky A. Assembly and mechanosensory function of focal contacts. Curr Opin Cell Biol 2001; 13:584 - 92; doi.org/10.1016/S0955-0674(00)00255-6 http://dx.doi.org/10.1016/S0955-0674(00)00255-6; PMID: 11544027
- Sabass B, Gardel ML, Waterman CM, Schwarz US. High resolution traction force microscopy based on experimental and computational advances. Biophys J 2008; 94:207 - 20; doi.org/10.1529/biophysj.107.113670 http://dx.doi.org/10.1529/biophysj.107.113670; PMID: 17827246
- Katsumi A, Orr AW, Tzima E, Schwartz MA. Integrins in mechanotransduction. J Biol Chem 2004; 279:12001 - 4; http://dx.doi.org/10.1074/jbc.R300038200; PMID: 14960578
- Millward-Sadler SJ, Salter DM. Integrin-dependent signal cascades in chondrocyte mechanotransduction. Ann Biomed Eng 2004; 32:435 - 46; http://dx.doi.org/10.1023/B:ABME.0000017538.72511.48; PMID: 15095818
- Schwartz MA. Integrins and extracellular matrix in mechanotransduction. Cold Spring Harb Perspect Biol 2010; 2:a005066; http://dx.doi.org/10.1101/cshperspect.a005066; PMID: 21084386
- Albinsson S, Nordström I, Hellstrand P. Stretch of the vascular wall induces smooth muscle differentiation by promoting actin polymerization. J Biol Chem 2004; 279:34849 - 55; http://dx.doi.org/10.1074/jbc.M403370200; PMID: 15184395
- Ono S, Abe H, Obinata T. Stimulus-dependent disorganization of actin filaments induced by overexpression of cofilin in C2 myoblasts. Cell Struct Funct 1996; 21:491 - 9; doi.org/10.1247/csf.21.491 http://dx.doi.org/10.1247/csf.21.491; PMID: 9078407
- Hayakawa K, Tatsumi H, Sokabe M. Actin stress fibers transmit and focus force to activate mechanosensitive channels. J Cell Sci 2008; 121:496 - 503; http://dx.doi.org/10.1242/jcs.022053; PMID: 18230647
- Hayakawa K, Tatsumi H, Sokabe M. Actin filaments function as a tension sensor by tension-dependent binding of cofilin to the filament. J Cell Biol 2011; 195:721 - 7; http://dx.doi.org/10.1083/jcb.201102039; PMID: 22123860
- Reusch HP, Chan G, Ives HE, Nemenoff RA. Activation of JNK/SAPK and ERK by mechanical strain in vascular smooth muscle cells depends on extracellular matrix composition. Biochem Biophys Res Commun 1997; 237:239 - 44; doi.org/10.1006/bbrc.1997.7121 http://dx.doi.org/10.1006/bbrc.1997.7121; PMID: 9268693
- Lee HS, Millward-Sadler SJ, Wright MO, Nuki G, Salter DM. Integrin and mechanosensitive ion channel-dependent tyrosine phosphorylation of focal adhesion proteins and beta-catenin in human articular chondrocytes after mechanical stimulation. J Bone Miner Res 2000; 15: 1501-9.; PMID: 10934648; DOI=10.1359/jbmr.2000.15.8.1501.
- Wang HB, Dembo M, Hanks SK, Wang Y. Focal adhesion kinase is involved in mechanosensing during fibroblast migration. Proc Natl Acad Sci U S A 2001; 98:11295 - 300; http://dx.doi.org/10.1073/pnas.201201198; PMID: 11572981
- Hornberger TA, Stuppard R, Conley KE, Fedele MJ, Fiorotto ML, Chin ER, et al. Mechanical stimuli regulate rapamycin-sensitive signalling by a phosphoinositide 3-kinase-, protein kinase B- and growth factor-independent mechanism. Biochem J 2004; 380:795 - 804; http://dx.doi.org/10.1042/BJ20040274; PMID: 15030312
- del Rio A, Perez-Jimenez R, Liu R, Roca-Cusachs P, Fernandez JM, Sheetz MP. Stretching single talin rod molecules activates vinculin binding. Science 2009; 323:638 - 41; http://dx.doi.org/10.1126/science.1162912; PMID: 19179532
- Sakai R, Iwamatsu A, Hirano N, Ogawa S, Tanaka T, Mano H, et al. A novel signaling molecule, p130, forms stable complexes in vivo with v-Crk and v-Src in a tyrosine phosphorylation-dependent manner. EMBO J 1994; 13:3748 - 56; PMID: 8070403
- Giannone G, Jiang G, Sutton DH, Critchley DR, Sheetz MP. Talin1 is critical for force-dependent reinforcement of initial integrin-cytoskeleton bonds but not tyrosine kinase activation. J Cell Biol 2003; 163:409 - 19; http://dx.doi.org/10.1083/jcb.200302001; PMID: 14581461
- Galbraith CG, Yamada KM, Sheetz MP. The relationship between force and focal complex development. J Cell Biol 2002; 159:695 - 705; http://dx.doi.org/10.1083/jcb.200204153; PMID: 12446745
- Jiang G, Giannone G, Critchley DR, Fukumoto E, Sheetz MP. Two-piconewton slip bond between fibronectin and the cytoskeleton depends on talin. Nature 2003; 424:334 - 7; http://dx.doi.org/10.1038/nature01805; PMID: 12867986
- Sawada Y, Nakamura K, Doi K, Takeda K, Tobiume K, Saitoh M, et al. Rap1 is involved in cell stretching modulation of p38 but not ERK or JNK MAP kinase. J Cell Sci 2001; 114:1221 - 7; PMID: 11228165
- Sawada Y, Tamada M, Dubin-Thaler BJ, Cherniavskaya O, Sakai R, Tanaka S, et al. Force sensing by mechanical extension of the Src family kinase substrate p130Cas. Cell 2006; 127:1015 - 26; doi.org/10.1016/j.cell.2006.09.044 http://dx.doi.org/10.1016/j.cell.2006.09.044; PMID: 17129785
- Puchner EM, Alexandrovich A, Kho AL, Hensen U, Schäfer LV, Brandmeier B, et al. Mechanoenzymatics of titin kinase. Proc Natl Acad Sci U S A 2008; 105:13385 - 90; http://dx.doi.org/10.1073/pnas.0805034105; PMID: 18765796
- Lange S, Xiang F, Yakovenko A, Vihola A, Hackman P, Rostkova E, et al. The kinase domain of titin controls muscle gene expression and protein turnover. Science 2005; 308:1599 - 603; http://dx.doi.org/10.1126/science.1110463; PMID: 15802564
- Baneyx G, Baugh L, Vogel V. Fibronectin extension and unfolding within cell matrix fibrils controlled by cytoskeletal tension. Proc Natl Acad Sci U S A 2002; 99:5139 - 43; http://dx.doi.org/10.1073/pnas.072650799; PMID: 11959962
- Hocking DC, Sottile J, McKeown-Longo PJ. Fibronectin’s III-1 module contains a conformation-dependent binding site for the amino-terminal region of fibronectin. J Biol Chem 1994; 269:19183 - 7; PMID: 8034677
- Gee EP, Ingber DE, Stultz CM. Fibronectin unfolding revisited: modeling cell traction-mediated unfolding of the tenth type-III repeat. PLoS One 2008; 3:e2373; http://dx.doi.org/10.1371/journal.pone.0002373; PMID: 19020673
- Cramer LP, Siebert M, Mitchison TJ. Identification of novel graded polarity actin filament bundles in locomoting heart fibroblasts: implications for the generation of motile force. J Cell Biol 1997; 136:1287 - 305; http://dx.doi.org/10.1083/jcb.136.6.1287; PMID: 9087444
- Balaban NQ, Schwarz US, Riveline D, Goichberg P, Tzur G, Sabanay I, et al. Force and focal adhesion assembly: a close relationship studied using elastic micropatterned substrates. Nat Cell Biol 2001; 3:466 - 72; http://dx.doi.org/10.1038/35074532; PMID: 11331874
- Ressad F, Didry D, Xia GX, Hong Y, Chua NH, Pantaloni D, et al. Kinetic analysis of the interaction of actin-depolymerizing factor (ADF)/cofilin with G- and F-actins. Comparison of plant and human ADFs and effect of phosphorylation. J Biol Chem 1998; 273:20894 - 902; http://dx.doi.org/10.1074/jbc.273.33.20894; PMID: 9694836
- De La Cruz EM, Sept D. The kinetics of cooperative cofilin binding reveals two states of the cofilin-actin filament. Biophys J 2010; 98:1893 - 901; doi.org/10.1016/j.bpj.2010.01.023 http://dx.doi.org/10.1016/j.bpj.2010.01.023; PMID: 20441753
- McGough A, Pope B, Chiu W, Weeds A. Cofilin changes the twist of F-actin: implications for actin filament dynamics and cellular function. J Cell Biol 1997; 138:771 - 81; http://dx.doi.org/10.1083/jcb.138.4.771; PMID: 9265645
- Galkin VE, Orlova A, Lukoyanova N, Wriggers W, Egelman EH. Actin depolymerizing factor stabilizes an existing state of F-actin and can change the tilt of F-actin subunits. J Cell Biol 2001; 153:75 - 86; http://dx.doi.org/10.1083/jcb.153.1.75; PMID: 11285275
- Wong DY, Sept D. The interaction of cofilin with the actin filament. J Mol Biol 2011; 413:97 - 105; doi.org/10.1016/j.jmb.2011.08.039 http://dx.doi.org/10.1016/j.jmb.2011.08.039; PMID: 21875597
- Galkin VE, Orlova A, Egelman EH. Actin filaments as tension sensors. Curr Biol 2012; 22:R96 - 101; doi.org/10.1016/j.cub.2011.12.010 http://dx.doi.org/10.1016/j.cub.2011.12.010; PMID: 22321312
- Kiyoshima D, Kawakami K, Hayakawa K, Tatsumi H, Sokabe M. Force- and Ca²⁺-dependent internalization of integrins in cultured endothelial cells. J Cell Sci 2011; 124:3859 - 70; http://dx.doi.org/10.1242/jcs.088559; PMID: 22100923
- Fournier MF, Sauser R, Ambrosi D, Meister JJ, Verkhovsky AB. Force transmission in migrating cells. J Cell Biol 2010; 188:287 - 97; http://dx.doi.org/10.1083/jcb.200906139; PMID: 20100912
- Doyle AD, Lee J. Cyclic changes in keratocyte speed and traction stress arise from Ca2+-dependent regulation of cell adhesiveness. J Cell Sci 2005; 118:369 - 79; http://dx.doi.org/10.1242/jcs.01590; PMID: 15632107
- Hotulainen P, Lappalainen P. Stress fibers are generated by two distinct actin assembly mechanisms in motile cells. J Cell Biol 2006; 173:383 - 94; http://dx.doi.org/10.1083/jcb.200511093; PMID: 16651381
- Ohashi K, Fujiwara S, Watanabe T, Kondo H, Kiuchi T, Sato M, et al. LIM kinase has a dual role in regulating lamellipodium extension by decelerating the rate of actin retrograde flow and the rate of actin polymerization. J Biol Chem 2011; 286:36340 - 51; http://dx.doi.org/10.1074/jbc.M111.259135; PMID: 21868383
- Grashoff C, Hoffman BD, Brenner MD, Zhou R, Parsons M, Yang MT, et al. Measuring mechanical tension across vinculin reveals regulation of focal adhesion dynamics. Nature 2010; 466:263 - 6; http://dx.doi.org/10.1038/nature09198; PMID: 20613844
- Wehland J, Osborn M, Weber K. Phalloidin-induced actin polymerization in the cytoplasm of cultured cells interferes with cell locomotion and growth. Proc Natl Acad Sci U S A 1977; 74:5613 - 7; http://dx.doi.org/10.1073/pnas.74.12.5613; PMID: 341163
- Pellegrin S, Mellor H. Actin stress fibres. J Cell Sci 2007; 120:3491 - 9; http://dx.doi.org/10.1242/jcs.018473; PMID: 17928305
- Menon S, Beningo KA. Cancer cell invasion is enhanced by applied mechanical stimulation. PLoS One 2011; 6:e17277; http://dx.doi.org/10.1371/journal.pone.0017277; PMID: 21359145