Figures & data
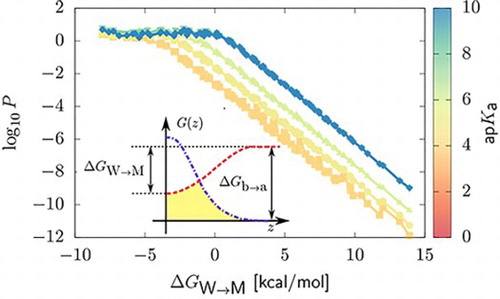
Figure 1. (a): we employ high-throughput coarse-grained simulations to screen a representative subset of the chemical space of small organic molecules. The systematic insertion of this large variety of compounds with very different polarities in a lipid bilayer results in an ensemble of PMFs, which dictate their permeability coefficient. (b): we highlight PMFs from the two main classes of compounds analyzed in this work: polar (top) and apolar (bottom). (c): Construction of the effective PMF (yellow area) contributing to the total resistivity
starting from the neutral (red) and charged (blue) PMFs of a compound. We highlight the main free-energy differences of the problem: the water membrane free-energy
of the neutral compound, and the acid/base free energy difference
dictating the shift between the two PMFs, directly linked to the ap
.
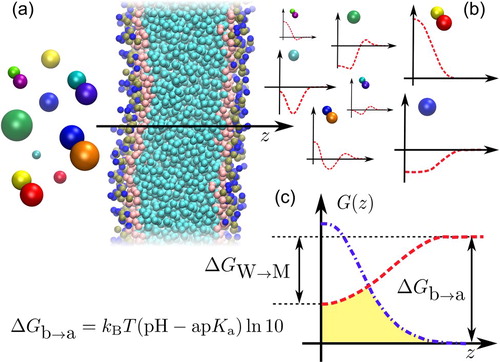
Figure 2. Two-dimensional projection of the permeability data on the plane in the case of neutral compounds and weak acids,
. Data are coloured according to the value of the ap
. Panels (a) and (b) distinguish between results obtained for Martini unimers and dimers. For neutral compounds with
, the permeability becomes independent on the value of the acid dissociation constant. Accordingly, we only show data up to
. We present permeability coefficients obtained from HTCG simulations (points) and the corresponding interpolated permeability profiles (lines). For neutral compounds, we include predictions from the Meyer-Overton rule in the presence of flat potentials of mean force with heights equal to
(‘Overton-mem’, dashed lines), see text.
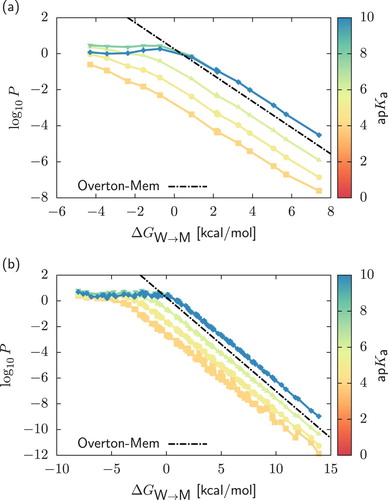
Figure 3. Construction of (blue) for an acidic compound from its charged
(red) and neutral
(orange) PMFs. In calculating
,
is vertically shifted by
, and we consider
. We present results for a polar (main plot) and an apolar (inset) dimer.
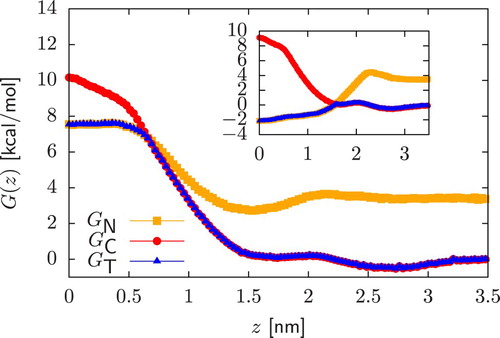
Figure 4. Main plot: Permeability data of CG dimers ( scale) calculated by relying on different CG representations of the deprotonated form of a compound. Starting from a neutral dimer, coloured points are obtained by assuming that deprotonation always occur in the chemical fragment encapsulated in the bead of higher polarity. In dashed lines (‘Apolar-dep’) we always deprotonate the bead of lower polarity. Inset: Comparison of the charged PMFs
arising from the two different CG representations of the deprotonated form of the neutral polar dimer in Figure . The PMF of the compound obtained by deprotonating the bead of lower polarity is characterised by a stronger repulsion.
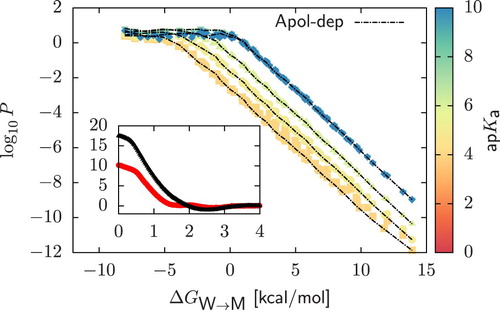
Figure 5. Panel (a): Two-dimensional projection of the permeability data on the plane in the case of strong acids with
. We include as reference a subset of the data obtained for weak acids,
. Points were obtained by assuming that starting from a neutral dimer, deprotonation always occur in the chemical fragment encapsulated in the bead of higher polarity. On the contrary, in dashed lines (‘Apolar-dep’) we always deprotonate the bead of lower polarity. Panel (b): Comparison of the neutral (main plot) and charged (inset) PMFs
and
of a
and a
dimers. In both cases, charged PMFs are obtained by assuming that deprotonation always occurs in the bead of higher polarity.
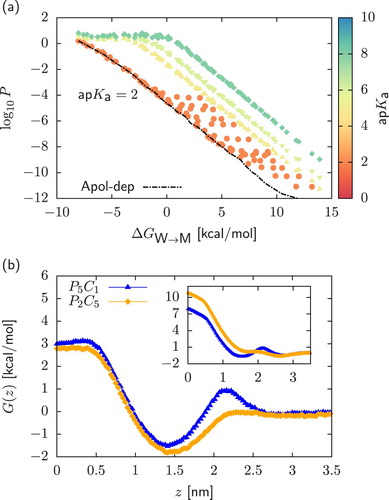
Figure 6. Permeability coefficients ( scale) for CG dimers in the case of strong acids with
. We display results as a function of
and the asymmetry
.
is calculated as the difference between the water/membrane partitioning free energy of the beads composing the dimer, sorting them in order of decreasing polarity. In all cases, the charged form of the compound was obtained by assuming that deprotonation always occur in the bead of higher polarity.
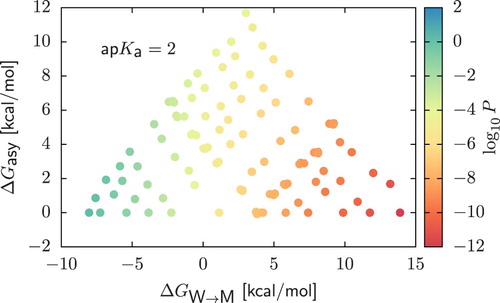