Figures & data
Figure 1. Sketch illustrating the assumed skin’s tissue microstructure. Skin’s tissue water is assumed to be entrapped within a cylindrical cavity that is attached to a spring-piston compound. The cavity’s wall represents the tissue’s elastic solid medium.
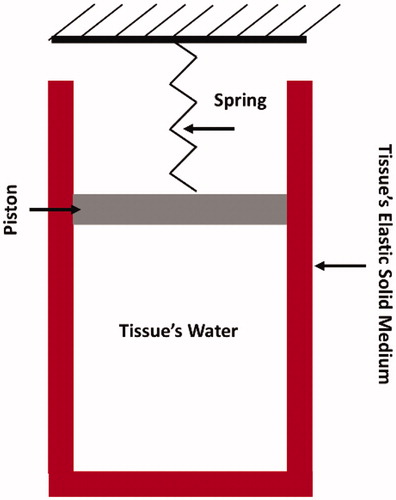
Table 1. Thermo-physical properties of skin tissue [Citation37].
Figure 2. The evolution of tissue’s relative volume (ratio of volume to initial volume), pressure, and temperature as a function of volumetric energy density in the tissue. The results are obtained using 10 J cm−2 pulse fluence, 20 µs pulse duration, and 3000 cm−1 absorption coefficient.

Figure 3. A sketch showing the discretization of the computational domain into N equal control volumes of size Δx. The dependent variables were solved at the spatial nodes placed in the center of the control volumes. Temperature profile between adjacent nodes was assumed to be linear.
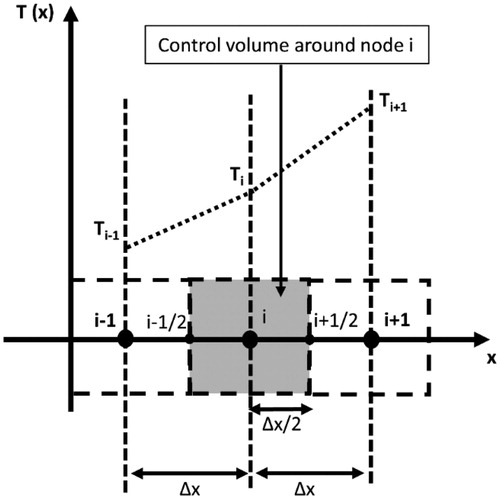
Figure 4. Verification of the computational model by comparing with the analytical model developed by Venugopalan et al. [Citation26]. The comparison is made in terms of (a) temperature profile outside the vapor-liquid layer, (b) thickness of the vapor–liquid layer, and (c) tissue’s ablation front position as a function of time. Continuous wave Er:YAG laser with irradiance (I = 150 W cm−2) was applied. Spatial and temporal grid size of 1 µm and 5 µs, respectively, were utilized.
![Figure 4. Verification of the computational model by comparing with the analytical model developed by Venugopalan et al. [Citation26]. The comparison is made in terms of (a) temperature profile outside the vapor-liquid layer, (b) thickness of the vapor–liquid layer, and (c) tissue’s ablation front position as a function of time. Continuous wave Er:YAG laser with irradiance (I = 150 W cm−2) was applied. Spatial and temporal grid size of 1 µm and 5 µs, respectively, were utilized.](/cms/asset/dfdf4db8-9ba3-4b99-872e-0332e1e3815e/ihyt_a_1620350_f0004_b.jpg)
Figure 5. Validation of the computational model by comparing with experimental results extracted from Ref. [Citation48]. The comparison is made in terms of tissue’s mass loss (ablation) as a function of pulse fluence. A pulse duration of 2 µs, a pulse frequency of 1 Hz, and an absorption coefficient of 400 cm−1 are employed here.
![Figure 5. Validation of the computational model by comparing with experimental results extracted from Ref. [Citation48]. The comparison is made in terms of tissue’s mass loss (ablation) as a function of pulse fluence. A pulse duration of 2 µs, a pulse frequency of 1 Hz, and an absorption coefficient of 400 cm−1 are employed here.](/cms/asset/dd8449dd-3487-4345-81db-456a245c64f9/ihyt_a_1620350_f0005_b.jpg)
Figure 6. Tissue temperatures as functions of time during and after a 200 µs long Er:YAG laser pulse at several selected depths within the tissue. The time is taken from the onset of irradiation. Pulse fluence is equal to 1.21 J cm−2. Surface tissue temperature calculated while neglecting water phase change is added for comparison.
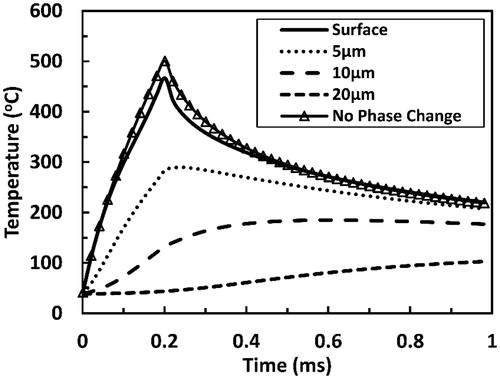
Figure 7. Temperature profiles predicted at several selected time points from the onset of laser tissue irradiation with a 200 µs long Er:YAG laser pulse. x represents the depth within the irradiated tissue with x = 0 indicating the surface. The pulse fluence is equal to 1.21 J cm−2.

Figure 8. Predicted coagulation thickness as a function of time as a result of irradiation with a 200 µs long Er:YAG laser pulse. Two sub-ablative fluence values (0.5 and 1 J cm−2) and one ablative fluence value (2 J cm−2) are presented. Ablation threshold here was 1.22 J cm−2.
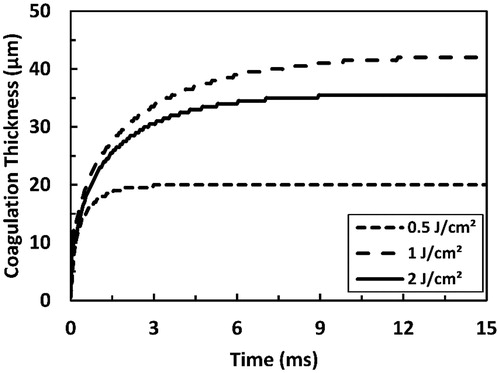
Figure 9. Predicted coagulation thickness as a function of the pulse fluence as a result of irradiation with Er:YAG laser pulse at several selected pulse durations (50, 500, and 1000 µs). The total simulation time is 15 ms.

Figure 10. Predicted coagulation thickness as a function of pulse duration as a result of irradiation with ER:YAG laser pulse at several selected pulse fluences. Fth refers to the pulse fluence that is equal to the ablation threshold at each pulse duration and the dashed line connects the points where the transition from sub-ablative to ablative conditions occurs. The total simulation time is 15 ms.
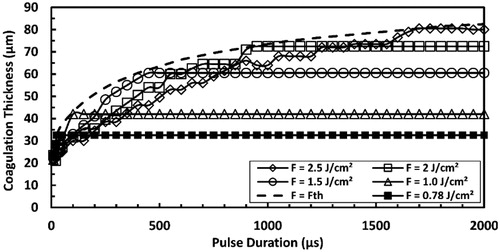
Figure 11. Predicted maximum coagulation thickness and ablation threshold at high strain-rate in comparison with those predicted at quasi-static loading for Er:YAG laser at pulse durations ranging from 10 µs to 2000 µs.
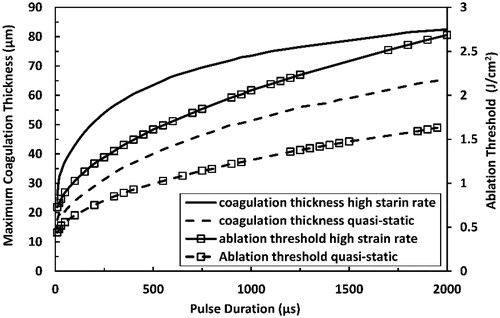
Figure 12. Predicted ablation efficiency as a function of both pulse duration and pulse fluence of a single Er:YAG laser pulse at (a) high strain-rate loading and at (b) quasi-static loading. Ablation efficency is the ratio between tissue-ablated mass and delivered laser energy.

Table 2. Comparison between model predictions and experimental observations in terms of pulsed laser tissue ablation outcomes’ values and trends.