Figures & data
Figure 1. Single-channel behavior of ClC-0. (a) Top trace: the two pores in ClC-0 are gated independently by the ‘fast gate’. The dashed line corresponds to zero current, and channel openings are downward deflections. Bottom five traces: Fifty seconds of continuous recording, shown on a compressed time scale. The long silent periods correspond to closures of the ‘common gate’. Recordings were at −70 mV; symmetric solutions contained 110 mM NMDG-Cl, pH 7.5 (J. Lisal and M. Maduke, unpublished). (b) Conditions similar to (a) except the extracellular pH has been raised to 8.5. The decrease in proton concentration causes an increased probability of common-gate closure. At this voltage (−70 mV), there is no significant effect of the extracellular pH change on the fast gate.
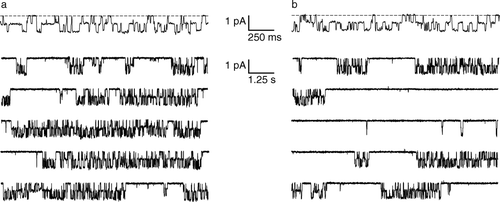
Figure 2. CLC structure. (a) Structures are shown from the point of view of the membrane. Above: ‘low resolution’ schematic diagram of CLC structure based on electrophysiological and biochemical studies of ClC-0. The CLC structure was predicted to be a two-pore homodimer, in which the pores are not perpendicular to the membrane. Below: cartoon view of the X-ray crystallographic structure of ClC-ec1 (pdb 1OTS). Each subunit of the homodimer contains two chloride ions (spacefilled in green). (b) Spacefilling view of the ClC-ec1 structure, focused on one of the subunits (shown in grey). Part of the protein has been peeled away to show the two chloride ions. E148, the ‘glutamate gate’ residue, blocks the upper chloride ion from exiting into the extracellular solution. S107 and Y445 directly coordinate the chloride ions, and S107 directly blocks the pathway for chloride to move between the two sites. (c) Close-up stereo view of the selectivity filter. S107, Y445, and E148 side chains are shown. (d) Close-up stereo view of the E148A mutant (pdb 1OTT). A chloride ion is seen in the place previously occupied by the glutamate side chain. The chloride pathway remains obstructed.
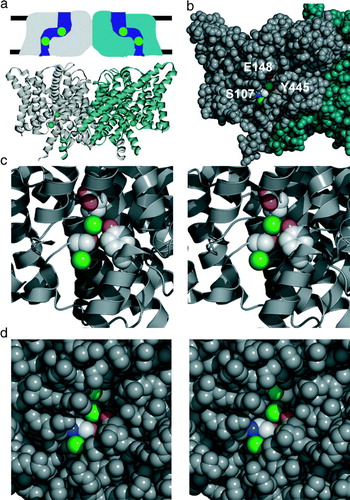
Figure 3. Model for chloride-proton antiport. In this minimal model, an electrochemical gradient for a transported ion is used to drive the cycle of conformational changes in a specific direction. In Step 1, two chloride ions bind from the extracellular side of the membrane. The binding of these chloride ions triggers a conformational change that allows a proton to bind to an intracellular site (step 2). The binding of this proton induces a conformational change that allows the chloride ions to exit from the intracellular side (step 3). The removal of chloride ions causes a conformational change allowing the transfer of protons from the intracellular side of the protein to the extracellular side of the protein, followed by the exit of protons to the extracellular side of the membrane (step 4). This cycle is repeated, allowing the coupled movement of two chloride ions and one proton in opposite directions. This simple model does not address the question of whether the two-subunit architecture (and the related ‘common’ gating seen in the CLC channels) is essential for CLC antiporter function.
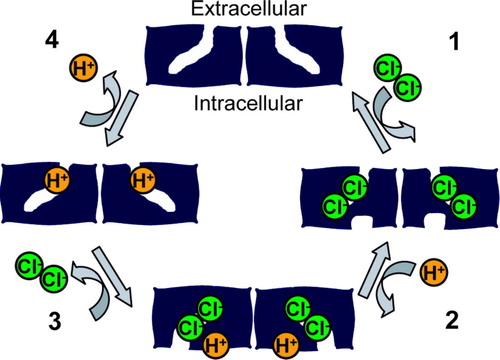
Figure 4. Gating asymmetry in ClC-0. (a) A single-channel record of ClC-0. Arrows indicate transitions of the common gate. Channel openings are downward deflections. There is an asymmetry in the gating cycle in that the common gate favors closing when 1 pore is opened and favors opening to a state with both pores open, as shown in this example. Conditions are the same as , except that the extracellular pH is 6.5 (J. Lisal and M. Maduke, unpublished). (b) State diagram indicating a net clockwise cycle from one-pore open (O1) to common-gate closed (inactivated, I) to both pores open (O2). The C to I transition is not measurable. (c) A model that can explain gating asymmetry in ClC-0. This speculative model illustrates that, by making one simple assumption, a channel with two gates can use the energy of a chloride electrochemical gradient to drive net cycling through its conformational states. Such cycling is similar to the cycling required for an antiporter to catalyze ion exchange (). In states O2, O1, and C, the common gate is open. In O2, the fast gates of both subunits are open. In C, the fast gates of both subunits are closed. In O1, the fast gate of only one of the subunits is closed. For simplicity, only one of the two ways of having one fast-gate closed is shown. In all three states, the pores transition very rapidly between different chloride-occupancy states (not all possible states are shown). When the fast gate is closed, chloride from the upper solution equilibrates with the sites in the pore. Channels can enter the inactivated state (I) (common gate closed) from C, O1, or O2. Only the transitions from O1 or O2 are measurable. By assumption, both gates can close only when there is no chloride in the pore. Fast-gate transitions occur during inactivation (substates a, b,c), but are not measurable. When there is a large electrochemical gradient for chloride (assume high chloride in the lower compartment), chloride saturates the binding sites as soon as the fast gate opens. This makes the transition from substate b to substate a essentially unidirectional, and leads to a net cycling in the clockwise direction (blue arrow). This figure is reproduced in colour in Molecular Membrane Biology online.
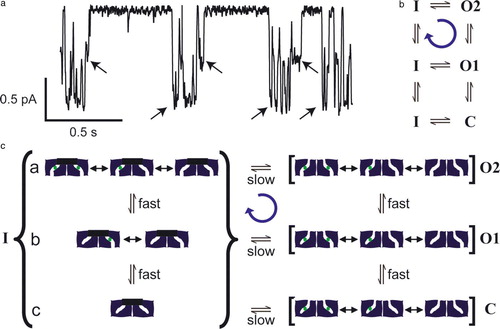