Figures & data
Figure 1. (S)Phase cyclins facilitate Cdk1-dependent phosphorylation of Cdc13 in vivo. (A) Measuring telomere length using teloblot: Loss of individual (S)phase cyclins show no change in telomere length (Compare lanes 1,2,17,18 to 3–6). Combined deletion of (S) phase cyclins, Δclb5Δclb6, result in shortened telomeres identical to the telomere phenotype observed in Cdc13 phosphorylation mutant, cdc13-T308A (Compare lanes 13,14 and 7,8 to 1,2,17,18). Additionally, cdc13-T308A along with Δclb5 or Δclb6 does not alter the short telomere phenotype observed in cdc13-T308A (Compare lanes 7,8 and 9–12). (B) Cell cycle dependent phosphorylation status of Cdc13(T308) in Δclb5Δclb6 vs WT: Haploid yeast strains carrying 3xFlag tagged Cdc13 with either WT (Top Panel) or Δclb5Δclb6 (bottom panel) was arrested in G1 using α-factor and released to progress through cell cycle collecting pellets every 15 min. Immunoprecipitation of Cdc13–3xFlag with anti-Flag in cell cycle synchronized yeast cells shows that there is a 30 min delay in Cdc13 phosphorylation in the strain lacking Clb5/6 compared with the WT. (C) FACS analysis indicative of the cell cycle progression after α-factor synchronization for strains used in ‘(B)’ shows that the onset of (S) phase is delayed by 30 min for Δclb5Δclb6 compared with WT.
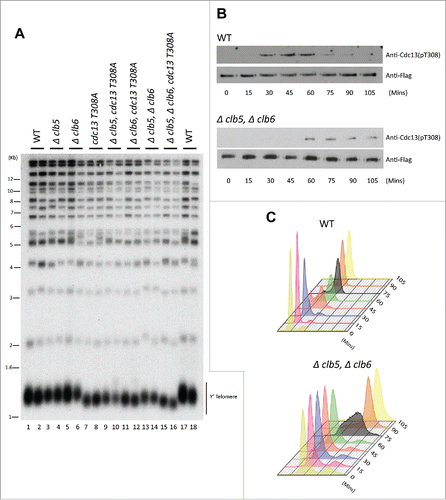
Figure 2. Clb5 and Clb6 are essential for the recruitment of Cdc13 and Est1 but not Stn1. Haploid yeast strains harboring 13xMyc tagged Cdc13, Stn1 or Est1 in a genetic background of WT or Δclb5Δclb6 was arrested in G1 using α-factor and released to progress through cell cycle collecting 15 min time points for the ChIP assay. ChIP was performed to evaluate the recruitment of Cdc13, Stn1 or Est1 in cell cycle synchronized WT vs Δclb5Δclb6 yeast cultures. (A) ChIP assay indicates that the cell cycle dependent recruitment of Cdc13 to telomeres is delayed and compromised in synchronous yeast cultures harboring Δclb5Δclb6 compared with WT. (B) FACS profile indicative of the cell cycle progression of synchronous yeast cultures used for the ChIP assay in ‘A’ - the onset of (S)phase is delayed by 30 min in Δclb5Δclb6 compared with WT. (C) ChIP assay indicates that the cell cycle dependent recruitment of Est1 to telomeres is delayed and compromised in cell cycle synchronized yeast cultures harboring Δclb5Δclb6 compared with WT. (D) FACS profile indicative of the cell cycle progression of synchronous yeast cultures used for the ChIP assay in ‘C’. (E) ChIP assay indicates that the cell cycle dependent recruitment of Stn1 to telomeres is delayed but not compromised in synchronous yeast cultures harboring Δclb5Δclb6 compared with WT. (F) FACS profiles indicative of cell cycle progression of synchronous yeast cultures used for the ChIP assay in ‘E’.
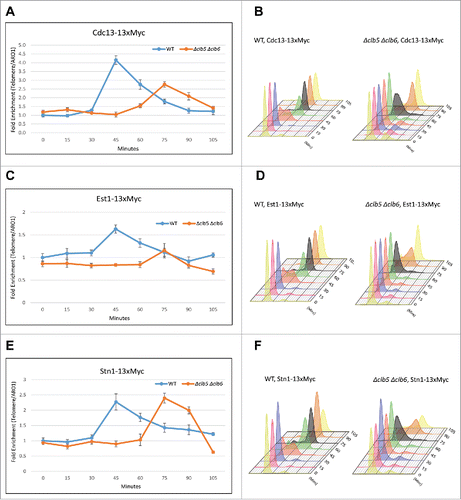
Figure 3. G2/(M)or M-phase cyclins do not alter telomere phenotype or the timing of Cdk1-dependent phosphorylation of Stn1 during cell cycle. (A) The first panel shows that telomere length measured for each of the genotypes, Δclb3, Δclb4, double mutant Δclb3Δclb4 or WT, indicates that the telomere length is unaltered in mutants lacking Clb3 and/or Clb4 compared with WT; the 2nd and 3rd panels show that loss of mitotic cyclins, Clb1 or Clb2 respectively, do not alter telomere length compared with WT. (B) Cdk1 dependent phosphorylation status of Stn1 for sites T223 and S250 were tested in yeast strains harboring deletions of different S- to M-phase cyclins compared with WT. Haploid yeast strains carrying 13xMyc tagged Stn1 with either WT (Top Panel) or Δclb5Δclb6 / Δclb3Δclb4 / Δclb2 (bottom 3 panels) was arrested in G1 using α-factor and released to progress through cell cycle with cultures collected every 15 min. Immunoprecipitation of Stn1–13xMyc with anti-Myc show no obvious difference in the profile of Stn1 phosphorylation detected with phospho-specific antibody Stn1-pS250 in synchronous yeast cultures harboring Δclb3Δclb4 / Δclb2 compared with WT. The phosphorylation of Stn1 in Δclb5Δclb6 coincides with the onset of S phase as shown in the FACS profile in ‘C’. (C) FACS profiles showing the cell cycle progression of the α-factor synchronized yeast cultures in ‘B’ – while loss of Clb5/6 delays the onset of S phase to 75 mins, neither Δclb3/4 nor Δclb2 cause variation to cell cycle progression compared with WT.
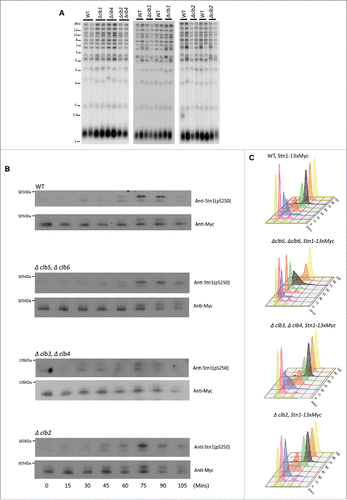
Figure 4. Clb2 but not Clb3/4 is responsible for the recruitment of Stn1 to telomeres in vivo. (A) ChIP assay indicates that the cell cycle dependent recruitment of Stn1 to telomeres shows small or no change in synchronous yeast cultures harboring Δclb3Δclb4 compared with WT. (B) ChIP assay indicates that the cell cycle dependent recruitment of Stn1 to telomeres is compromised in synchronous yeast cultures lacking Clb2 compared with WT. (C) FACS profiles indicative of cell cycle progression in synchronous cultures used in ‘A’ and ‘B’. (D) ChIP assay in asynchronous yeast cultures with 13xMyc tagged Cdc13 indicates that the recruitment of Cdc13 to telomeres is unaffected in Δclb3Δclb4 or Δclb2 compared with WT.
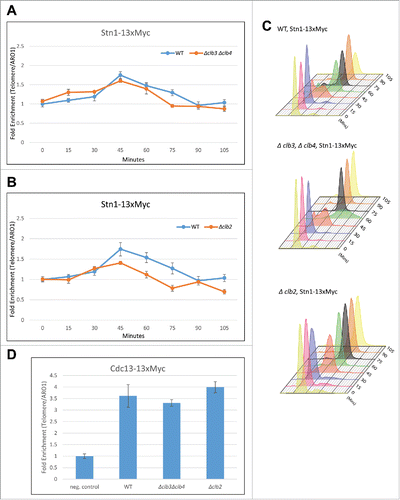
Figure 5. Clb2 facilitates the phosphorylation of Stn1 in vitro while Cdc13 phosphorylation lacks significant specificity for any particular Cyclin-Cdk1 complex in vitro. (A) TAP-tagged Cyclins were purified in yeast strains harboring Cdk1-as1 allele. The silver stained gel shows the different Cyclin-Cdk1-as1 complexes purified through TAP-tag purification. *, Clb5/Clb3/Clb2. (B) In vitro Kinase assay analyzing the differential phosphorylation efficiency of Stn1(M) by different Cyclin-Cdk1-as1 complexes. The top panel shows the coomassie blue stained input, purified 6xHis tagged recombinant Stn1(M), WT and stn1-T223A, S250A. The bottom panel shows the corresponding autoradiograph from the kinase assay. (C) Quantification of the corresponding phosphorylation efficiency (WT/mutant) obtained from the autoradiograph over input in ‘B’ shows that Clb2-Cdk1-as1 phosphorylates Stn1(M) with greatest efficiency followed by Clb3-Cdk1-as1 and finally Clb5-Cdk1-as1. (D) In vitro Kinase assay analyzing the differential phosphorylation efficiency of Cdc13 by different Cyclin-Cdk1-as1 complexes. The top panel shows the coomassie blue stained input, purified 6xHis tagged recombinant Cdc13, WT and mutant (T308A). The bottom panel shows the corresponding autoradiograph for the kinase assay. (E) Quantification of the corresponding phosphorylation efficiency (WT/mutant) obtained from the autoradiograph over input in (D) shows no significant specificity of any particular Cdk1-cyclin for phosphorylation of Cdc13 in vitro.
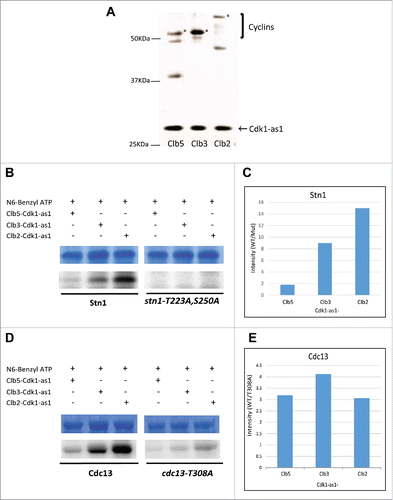
Figure 6. Model showing the mechanism of sequential phosphorylations of Cdc13 and Stn1 during cell cycle progression and their role in telomere elongation. S-phase cyclins Clb5/6 initiate Cdk1-dependent phosphorylation of Cdc13 at T308 which promotes the recruitment of Cdc13 and thereby Est1 to telomere, leading to telomere elongation. This is subsequently followed by the sequential phosphorylation of Stn1 at sites T223 and S250 by Cdk1, facilitated by Clb2. The phosphorylation of Cdc13 during G2/M is potentially antagonized in vivo, by phosphatase, perhaps Cdc14, which is involved in dephosphorylating Cdk1 targets (indicated by blue “??”). Thus, the ordered phosphorylation of CST components by different cyclin-Cdk1 complexes, perhaps in combination with phosphatases, regulates telomerase-extendable and CST-unextendable states of the telomere.
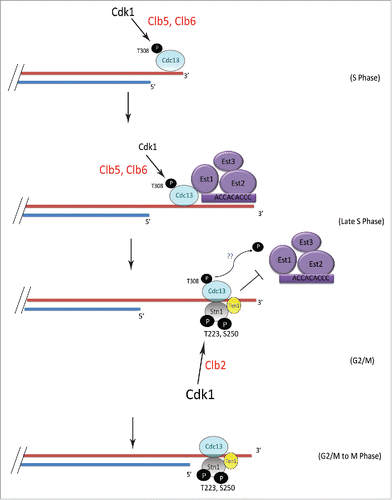