Figures & data
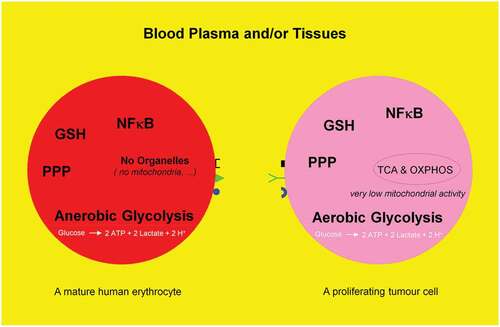
Figure 1. Role of oncogenes, tumor suppressor genes, and their corresponding proteins in tumorigenesis and metabolic switch. (a) A well-balanced expression of oncogenes and tumor suppressor genes, as well as a well-balanced activity of oncogenes and tumor suppressor proteins reciprocally controlling each other constitute the prerequisite of a normally functioning cell. Dependent on extracellular stimuli (e.g. glucose uptake) the synthesis and activity of these proteins are transiently activated or repressed. (b) However, mutations promoting synthesis and activities of oncoproteins or curbing synthesis and activities of tumor suppressor proteins enable the differentiated cell to turn into a undifferentiated tumor cell, thus introducing the metabolic switch, resulting in unlimited growth and proliferation.
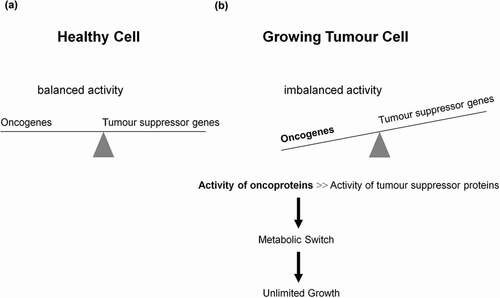
Figure 2. Schematic representation of anaerobic glycolysis, oxidative phosphorylation (OXPHOS/respiration), and aerobic glycolysis/Warburg effect. (a) Anaerobic glycolysis in anucleated and organelle-free human and mouse erythrocytes and conversion of glucose to lactate. Most differentiated cells convert glucose to pyruvate via glycolysis and subsequently to CO2 via TCA and OXPHOS, with marginal lactate production. (b) Proliferating cells prefer aerobic glycolysis, i.e. marginal amount of pyruvate is dispatched to mitochondrion and simultaneously high amounts of lactate are generated.
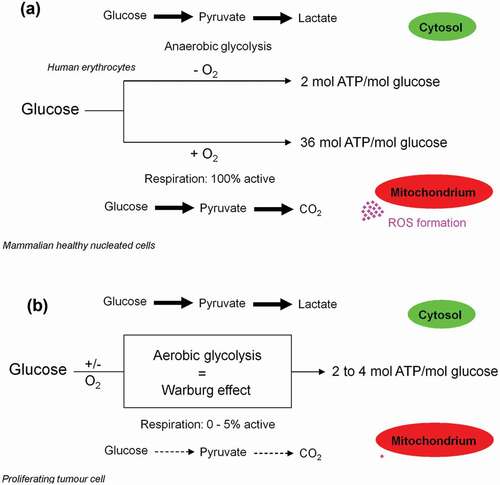
Figure 3. Glucose uptake and internalization by glucose transporters (GLUTs) and its phosphorylation at C6-position via the glycolytic enzyme hexokinase. Glycolysis implies conversion of glucose 6-phosphate (G6P) into lactate generating only two ATP molecules per one molecule of glucose. G6P can equally be entered into the pentose phosphate pathway (PPP), consisting of irreversible oxidative and reversible non-oxidative branches. The irreversible dehydrogenase/decarboxylase system of the oxidative branch of the PPP (ox-PPP) consisting of glucose 6-phosphate dehydrogenase (G6PDH), 6-phosphogluconate dehydrogenase (6PGD) and 6-phosphogluconolactonase (6PGL) break down G6P, yielding two NADPH and one ribulose-5-phosphate (Ru5P). Consequently, the two non-oxidative systems of PPP takes on the remaining work. The isomerizing/epimerizing system consisting of RPI and ribulose 5-phosphate 3-epimerase (RPE), interconverts Ru5P to xylulose 5-phosphate (X5P) and ribose 5-phosphate (R5P), whereas the sugar rearrangement system consisting of transketolase (TK) und transaldolase (TA), interconverts X5P and R5P to the glycolytic intermediates fructose 6-phosphate (F6P) und glyceraldehyde 3-phosphate (GAP). Thus, the PPP culminates in glycolysis. Furthermore, F6P and GAP can in turn be converted into R5P based on the reversible nature of the non-oxidative branch of the PPP (non-ox-PPP) .
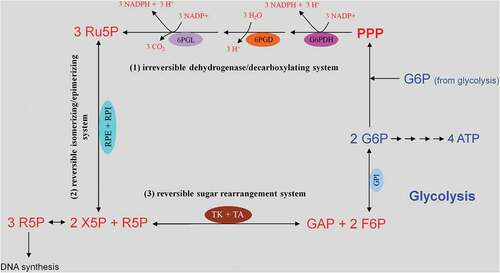
Figure 4. Dependency of growing tumor cells, activated T- and proliferating cells on glycolysis. The metabolic shift from respiration to glycolysis, known as the Warburg effect in proliferating tumor cells, is not solely restricted to them. For instance, activated T- and proliferating cells equally make use of the same mechanisms. The six hallmarks of a proliferating tumor cell are: high activities of glycolysis and pentose phosphate pathways, massive glutaminolysis and fatty acids biosynthesis, as well as angiogenesis and metastasis. However, the latter two features do not appear in healthy activated T- and proliferating cells.
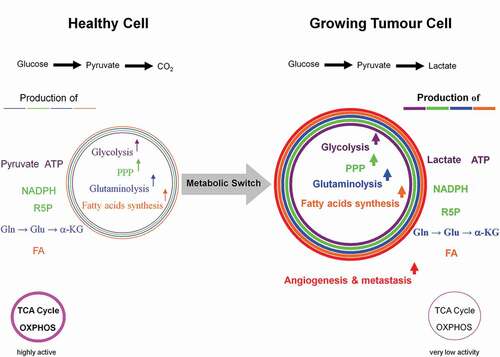
Figure 5. Coordinated interactions between glycolysis, citrate cleavage enzyme, and glutaminolysis for the fatty acids biosynthesis in growing tumor cells. Based on the very low activity of the TCA cycle in growing tumor cells, glucose is diverted away from mitochondrial acetyl-CoA and citrate production. Alternatively, glutamine-derived α-ketoglutarate (α-KG) and its subsequent reductive carboxylation by NADPH-dependent isocitrate dehydrogenase 2 (IDH2) results in citrate production. The latter is then converted to acetyl-CoA and oxaloacetate by the citrate cleavage enzyme (CCE). This extramitochondrial acetyl-CoA is used for long chain fatty acids biosynthesis. The enzymic decarboxylation of oxaloacetate by the cytosolic phosphoenolpyruvate carboxykinase 1 (PEP-CK1) results in phosphoenolpyruvate (PEP) formation which finally is primarily converted into lactate which leads to tumor acidic microenvironment. Marginal amount of pyruvate is also dispatched to mitochondrion. The oxaloacetate-to-malate conversion by NADH-dependent malate dehydrogenase (MDH) and the subsequent malic enzyme mediated decarboxylation of malate into pyruvate maintain the regeneration of NAD+ and a continual supply of NADPH, respectively. NAD+ regeneration ensures the perpetuation of the glycolysis pathway, whereas the generation of NADPH supports lipogenesis. The energy-linked nicotinamide nucleotide transhydrogenase (Nnt) acts as an effective buffer system and catalyze the direct transfer of a hydride ion between NADH and NADPH. The interconnection between the NADPH consuming reductive carboxylation and NADPH producing oxidation of α-KG is also demonstrated.
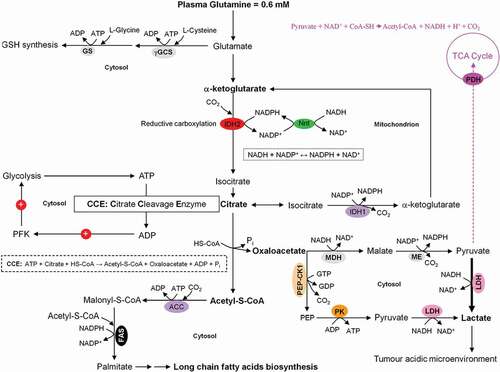
Figure 6. Metabolism consisting of catabolic and anabolic processes encompasses the sum of all chemical conversions in a cell or organism. (a) Anabolism and (b) catabolism.
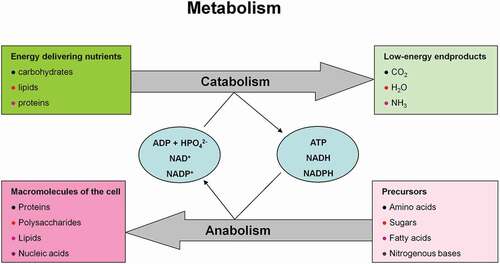
Figure 7. Anaerobic and aerobic glycolysis in mature human erythrocytes and growing tumor cells. (a) In both cases, the rate of the glycolytic ATP production is the same (Glucose + 2ADP → 2 Lactate + 2H+ + 2ATP). (b) For adequate supply of the organism with molecular oxygen, human erythrocytes divert 20% of the uptaken glucose to Rapoport and Luebering glycolytic shunt [Citation102]. This carries an energetic cost based on bypassing the ATP generating phosphoglycerate kinase (PGK). (c) Besides 2,3-bisphosphoglycerte (2,3-BPG) synthase/2-phosphatase (BPGM), multiple inositol polyphosphate phosphatase (MIPP1) is also able to significantly decrease 2,3-BPG levels in vivo.
![Figure 7. Anaerobic and aerobic glycolysis in mature human erythrocytes and growing tumor cells. (a) In both cases, the rate of the glycolytic ATP production is the same (Glucose + 2ADP → 2 Lactate + 2H+ + 2ATP). (b) For adequate supply of the organism with molecular oxygen, human erythrocytes divert 20% of the uptaken glucose to Rapoport and Luebering glycolytic shunt [Citation102]. This carries an energetic cost based on bypassing the ATP generating phosphoglycerate kinase (PGK). (c) Besides 2,3-bisphosphoglycerte (2,3-BPG) synthase/2-phosphatase (BPGM), multiple inositol polyphosphate phosphatase (MIPP1) is also able to significantly decrease 2,3-BPG levels in vivo.](/cms/asset/5b5b829f-7b32-4f06-8772-2f5ae1778c7f/kccy_a_1618125_f0007_oc.jpg)
Figure 8. Role of the pentose phosphate pathway (PPP) in cellular defense and DNA synthesis. (a) In the oxidative branch of the PPP (ox-PPP), glucose 6-phosphate (G6P) is converted in three consecutive reactions into ribulose 5-phosphate (Ru5P), simultaneously yielding two NADPH molecules. (b) Linkage of glutathione (GSH) cycle to NADPH producing PPP provides cellular defense mechanisms against oxidative stress by glutathione reductase (GR) and -peroxidase dependent (GPx) detoxification of lipid peroxides (ROOHs) and hydrogen peroxide (H2O2). (c) In the non-oxidative branch of the PPP (non-ox-PPP), the isomerizing system interconverts Ru5P to xylulose-5-phosphate (X5P) and R5P. In the sugar rearrangement system transketolase (TK) which transfers two carbon units and transaldolase (TA), which transfers three carbon units, convert X5P and R5P into the glycolytic intermediates fructose-6-phosphate (F6P), and glyceraldehyde-3-phosphate (GAP). (d) The generated NADPH molecules are also required for and consumed during nucleotides biosynthesis. NADPH links PPP with the electron transmitting systems namely, GSH-glutaredoxin(Grx) and thioredoxin (Trx). The oxidation of NADPH channels its hydride ion (H −) to these hydrogen carrier systems which serve as electron (H −) donors. R5P conversion to phosphoribosyl pyrophosphate (PRPP), as well as purine serve as the backbone for ribonucleotides synthesis. Reduction of ribonucleotide diphosphate (rNDP) to deoxynucleotide diphosphate (dNDP) and its phosphorylation by nucleotide diphosphate kinase (NDK) is an absolute prerequisite for DNA synthesis.
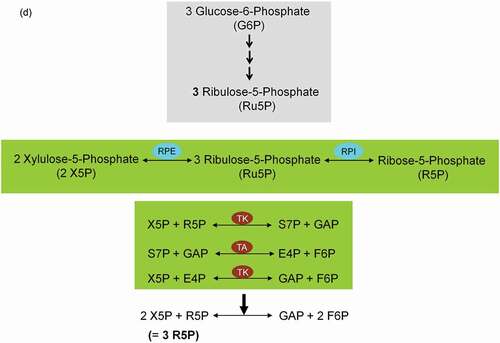
Figure 9. Intercross between glycolysis-, sorbitol-, pentosephosphate, and glyoxalase pathways in mature human erythrocytes. For more details see the main text.
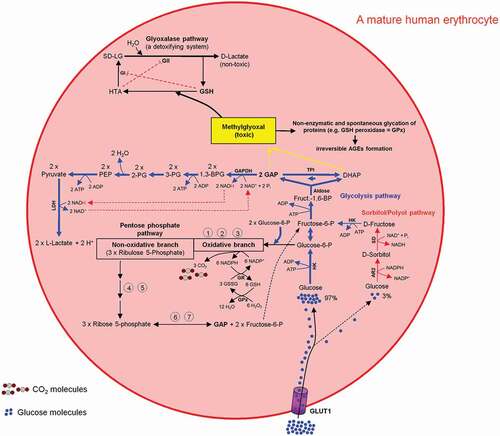
Figure 10. Intercross between glycolysis-, sorbitol-, pentosephosphate, and glyoxalase pathways in mammalian cells especially in a proliferating tumor cell. For more details see the main text.
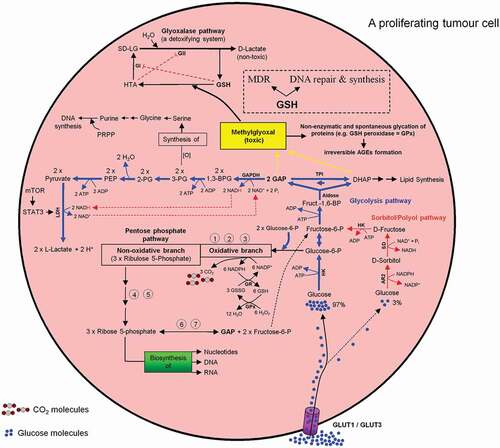
Figure 11. Association between high glycolytic flux and extracellular pH (pHe) gradient formation by carbonic anhydrase, as well as proton pump and exchangers. Glycolysis-associated lactate generation promotes hypoxia-inducible factor-1a (HIF-1α) activation and HIF-1α dependent transcription of several proton channels and exchangers. Extrusion of protons (H+) by these regulators contributes to a concomitant extracellular acidification (pHe: 6.8) and intracellular alkalinization (pHi: 7.2) in growing tumor cells. Additional source of tumor microenvironment acidity is the activity of carbonic anhydrase IX (CAIX). CAIX-dependent hydration of carbon dioxide (CO2) – predominantly generated by the oxidative branch of the pentose phosphate pathway (ox-PPP) and to a lesser extent by TCA cycle – delivers protons (H+) and hydrogen carbonate (HCO3−) ions. Subsequent uptake of HCO3− by Na+-dependent bicarbonate (NBC) transporter and anion exchanger 2 (AE2), replenishes the intracellular HCO3−. Titration of HCO3− by intracellular H+ ions, produced by glycolysis, results in the formation of CO2 which rapidly diffuses across the plasma membrane, where it meets CAIX. MCT: monocarboxylate transporter, V-ATPase: vacuolar-type proton ATPase, NHE-1: sodium/hydrogen exchanger 1, AE2: anion exchanger 2, NBC: sodium/bicarbonate (Na+/HCO3−) co-transporter, CAIX: carbonic anhydrase 9.
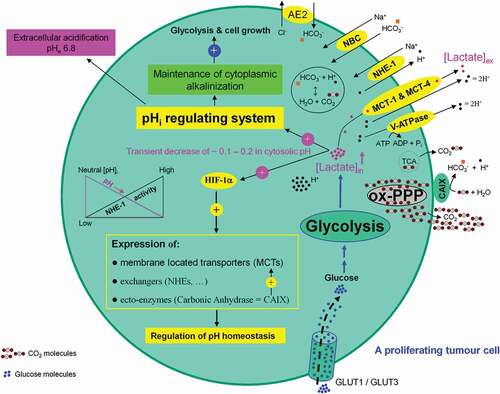
Figure 12. (a) Inverse correlation between aerobic glycolysis and respiration in a proliferating tumor cell. (b) Positive and dynamic interaction between glycolysis and pentose phosphate pathway (PPP). (c) Mitochondrial respiratory chain (MRC) the main source of superoxide anion (O2●−) generation.
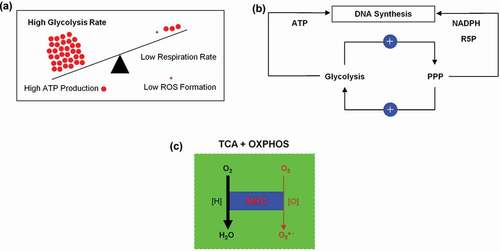