Figures & data
Figure 1. Simplified view of the thiamine biosynthesis pathway in E.coli. Enzymes encoded by the thiC operon (thiC and thiF,S,G,H, dashed box) synthezise the primary substrates hydroxymethylpyrimidine phosphate (HMP-P) and hydroxyethylthiazole phosphate (HETP). AIR: Amino-imidazole ribotide. Note that thiE is in the same operon. Genes in the thiM operon (thiM and thiD in gray) code for kinases that ultimately produce HMP-PP. Adapted from.Citation3
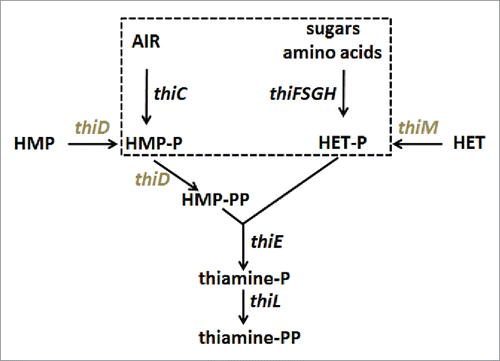
Figure 2 Organization, regulation mechanisms and secondary structures of E.coli and A.thaliana thiamine pyrophosphate riboswitches. (A) In E.coli, riboswitches are located in the 5′-UTR and control translation (thiM, shown here as an example) and/or transcription (thiC) of the downstream genes. At low TPP concentration, the aptamer (black) is not fully folded and the regulatory sequence (here the Shine-Dalgarno sequence,SD) in the expression platform (EPF, gray) is available for gene expression whereas, at higher concentration, TPP binding induces a sequestering of SD, which prevents initiation of translation see for thiC. (B) In A.thaliana, the TPP riboswitch is located in the 3′-UTR of the gene THIC. At low TPP concentration, the 5′-strands of helices P4 and P5 pair within intron 2 (In2), which masks the 5′-splice site, leaves the major poly-adenylation site available and produces a short stable transcript. At higher TPP concentration, the riboswitch aptamer folds completely, which permits Intron 2 splicing and removal of the major poly(A) site thus producing a longer unstable transcript (adapted from Citation12). (C) Structure of the ditopic thiamine pyrophosphate with the pyrimidine and pyrophosphate groups interacting at different locations within the RNA (see D). (D) The sequences of the bacterial riboswitch aptamers are the wild type sequences, whereas the sequence of ATthiC is the one used for the crystallographic study.Citation51 The stem P3 is most variable in plant riboswitches and the retained shortened P3 corresponds to the conserved part.Citation12 The box labeled ECthiCmutP1 corresponds to the sequence of a P1-helix variant of ECthiC (see text). The conserved loop sequence UGAGA between P2 and P3 is the binding site for the pyrimidine moiety of the TPP and the conserved internal loop between P4 and P5 is the pyrophosphate binding site.
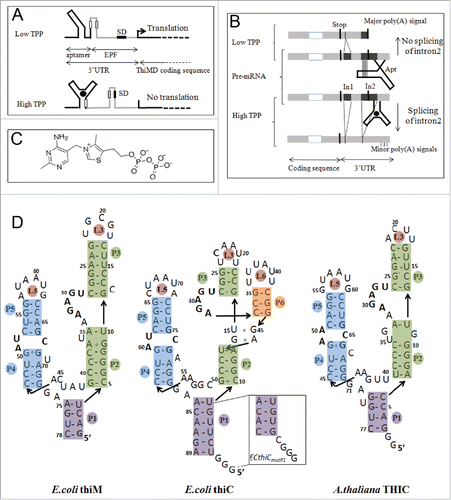
Figure 3. For figure legend, see next page.Figure 3. (figure previous page) Kinetics of ATthiC cleavage from •OH footprinting. (A) Each color corresponds to a particular time after TPP addition from t = 0 (pure red), to t = 30 min (pure blue). The times were 0, 1, 2.5, 5, 7.5, 10, 13, 16, 20, 28, 46, 95, 200, 400, 800, 1000, 1200, 1400, 1600 and 1800 s. Conditions: TPP 2 µM, magnesium acetate 2 mM, sodium cacodylate buffer 5 mM, pH 6.5, 25 °C. The fact that no cleavage variation was observed from G17 to G24, which was not imposed in the processing, is a good indication of the quality of the overall quantification procedure since these residues form a quickly folded apical loop not susceptible to be influenced by TPP binding. Cleavage curves for selected residues are shown in . A typical gel is shown in Fig. SD-3D. The inset shows the correlation of each cleavage curve with the cleavage curve for t = 0 as a function of time, which highlights the biphasic character of the kinetics. (For this correlation curve, the experimental data in the main figure, limited to the residue range U12-G54, were supplemented with other data to cover the wider residue range U12-G69).The solid curve is from a bi-exponential fit; the resulting times τshort and τlong from the fit correspond well with those obtained for individual cleavage curves in . (B) Kinetics of •OH cleavage for selected residues of ATthiC. The experimental points are those for six particular residues (U12, U16, C26, A29, A43 and G69) from and from other data (not shown). A few experimental points were flagged as outliers and suppressed (e.g., t = 1 s for A29 and A43). The two kinds of fit mentioned in the text are shown: in blue, the simple bi-exponential fit (equation Equation4(2b)
(2b) ) and, in red, the fit making use of kinetic model #1 proved to be the correct one in the following (see Fig. SD-10 for an assessment of the quality of fit). The times
and
reported for each residue result from the bi-exponential fit and are related to
and
in equation (Equation4
(2b)
(2b) ) by
and
.
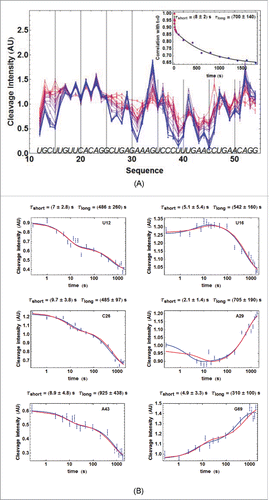
Table 1. Comparison of SPR and •OH results on ATthiC for model #1. The indicated values of (from SPR or ITC) were used to constrain the search of the kinetic parameters with the •OH cleavage curves (see text). The value for
is the value estimated at 25°C for the major RNA species representing ca. 95 % of the active RNA: Fig. SD-17A,C.
Table 2. Confrontation of the two models with SPR and kinITC results on ECthiCmutP1. The values from SPR were obtained at 25°C, and those from kinITC were calculated for 25°C from the results obtained at 20, 27, 30, 34 and 37°C. Only model #1 led to excellent agreement for from the two techniques, whereas model #2 led to serious disagreement for
and considerable disagreement for
As already mentioned in Citation22 for model #1, both kinetic models and both techniques disagree on
by a factor of 6. However, only kinITC is in agreement with the very well determined value of
obtained by ITC after independent measurements at five temperatures.
Figure 4. Thermodynamic and kinetic results from kinITC for ECthiM. (A) Thermodynamic results. The evolution with the temperature of the thermodynamic parameters of all steps in equations 1a,b was obtained with kinITC used with model #1. The figures for ΔG and ΔS refer to the complete reaction involving TPP binding and RNA folding. The figure for ΔH collects ΔHBinding, ΔHFolding (dashed curve) and ΔHITC = ΔHBinding + ΔHFolding shown in blue with the individual values obtained by normal use of ITC at five different temperatures (blue dots). Note the extremum of Kd reached at the temperature where ΔHBinding = 0 in agreement with the Van't Hoff equation linking Kd(binding) and ΔHBinding. This extremum is here a maximum because is positive. The injection curves of the kinITC experiments at different temperatures and their fit are shown in Fig. SD-5C. (B) Kinetic results. The evolution with the temperature of all kinetic parameters in equations 1a,b was obtained with kinITC used with model #1. The activation energies and
were obtained as adjustable parameters in kinITC, and
and
were deduced from
and
, respectively.
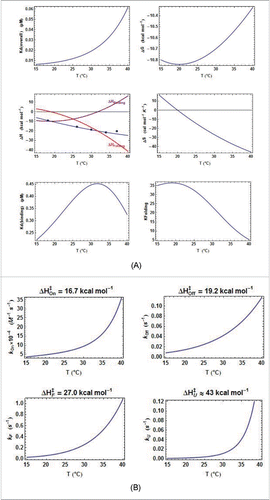
Figure 5. Comparison of the thermodynamic parameters for E. coli riboswitches. A. Comparison of all ΔH terms from kinITC. The three curves are related by ΔHITC= ΔHBinding+ ΔHFolding. The pattern is similar for the two E. coli riboswitches: the two ΔHBinding curves cross ΔHBinding= 0 at nearby temperatures and the two ΔHFolding curves are quasi parallel. B. Quasi absence of temperature variation for Each
curve has been normalized to a unit value at its maximum (0.93 µM for ECthiC and 0.45 µM for ECthiM).
is almost constant for ECthiC and varies at most twofold for ECthiM in a broad temperature range in comparison of
which varies tenfold for ECthiM () and more than thirtyfold for ECthiC (Fig. SD-7A).
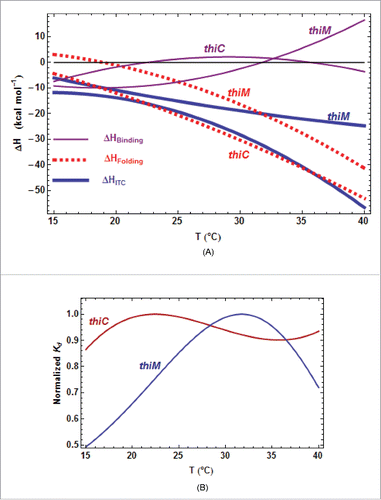
Figure 6. Primary TPP/RNA interaction for ATthiC. (A) The evolution along the sequence of the normalized difference (see text) is shown. A large negative (resp. positive) value of the difference is the mark of early protection against cleavage (resp early enhanced cleavage) after TPP addition. The red lines mark the positive and negative limits at a 5σ threshold. B. The closed riboswitch from the crystal structure has been opened to visualize the primary interaction of the TPP with the RNA; only the pyrophosphate of the TPP is visible (arrow). The loops L5 and L3 are labeled (). The colors evolve from pure blue to pure red for, respectively, the most negative and most positive values of the normalized difference
shown in . The greenish color corresponds to the 5′-end and 3′-end residues for which no accurate results were obtained. All significantly negative values (pure blue), as well as the highest positive values (pure red), are exclusively located around the interaction site of the TPPpyrimidine moiety.
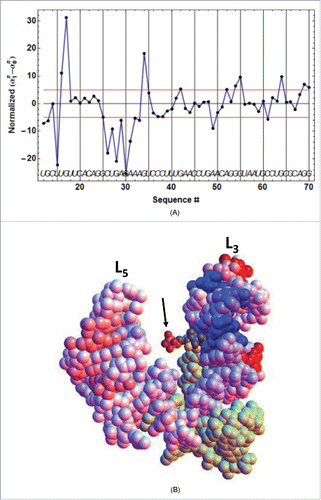
Figure 7. TPP concentration dependence of the kinetic regulation by ECthiC and ECthiM. The dependence of on the TPP concentration from equation (3) is shown (solid curves) for a common temperature (37°C) and for three values of
. The value for each middle curve (9 s for ECthiC and 12 s for ECthiM ) corresponds to the experimental pausing time observed at 37°C with NusA. The vertical bar on each middle curve marks the inflection point where the regulation efficiency is maximum. The two other curves corresponding to half and twice the pausing time are shown to illustrate the effect of this parameter on the regulation. The black dashed curves correspond to
that would be obtained if each riboswitch was thermodynamically, and not kinetically controlled, which emphasizes the strong kinetic character of the regulation.
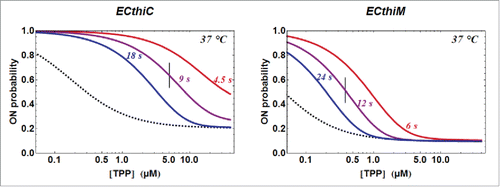
Figure 8. RNA polymerase pause sites observed during ECthiC template transcription. (A) Transcription kinetics without nusA (see SD-15AB). Transcription times are indicated on top of the gel. Pause sites positions are indicated on the right (FL: full length transcript). The leftmost lane is an RNAse-T1 ladder. Significant pausing is visible at upstream positions of C135. Transcription was performed at 30°C. (B) Transcription kinetics with NusA (0.3 µM). Pausing at C135 is now prominent as compared to A. Transcription experiment was performed at 37°C. (C) Sequence upstream of the pausing site at C135. The footprint of the polymerase is indicated by the gray rectangle. The hairpin structure forms a class I pause site according to.Citation39 (D) Kinetic analysis of pausing at C135. The exponential time τ = 8.4 s corresponds to the average pausing time. The points corresponding to 1 and 5 s (in gray) were not used in the fit.
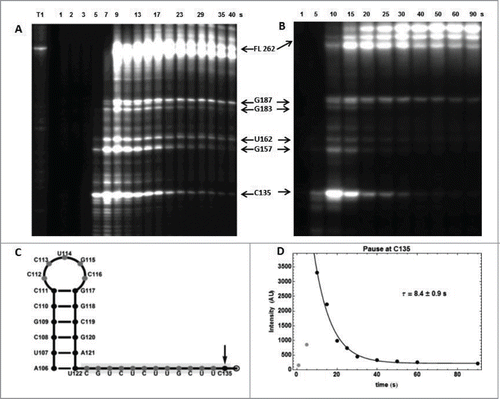