Figures & data
Figure 1. Schematic representation of stimuli-responsive delivery of therapeutics and contrast agents into a diseased cell. After entering a targeted cell through endocytosis, nanoparticles undergo disassembly through various internal (acidity, actions of intracellular enzymes, high temperature) as well as external (light, magnetic field, temperature, ultrasoud, etc.) stimuli. On dissociation of the nanostructure, the encapsulated drugs or imaging agents are released and delivered to their specific targets in nucleus and cytoplasm
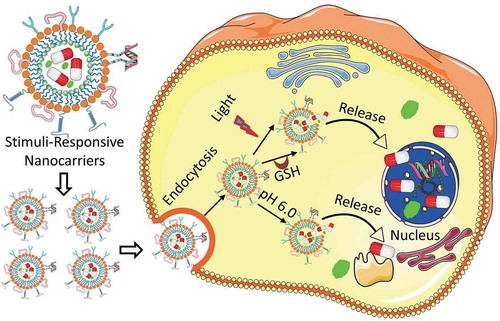
Figure 2. A multifunctional nanocarrier-based drug delivery system containing targeting moieties (antibody, peptide, aptamer, etc.) and active components (drug, nucleic acid, protein, contrast agent, etc.). Drugs and imaging agents are loaded in the core structure (or membrane) of the multifunctional nanocarrier, whereas the targeting moieties such as antibody, peptide, aptamer, etc. and nucleic acids in most cases are conjugated on the surface of the nanostructure through various linkers, electrostatic interactions as well as covalent or noncovalent bond formation
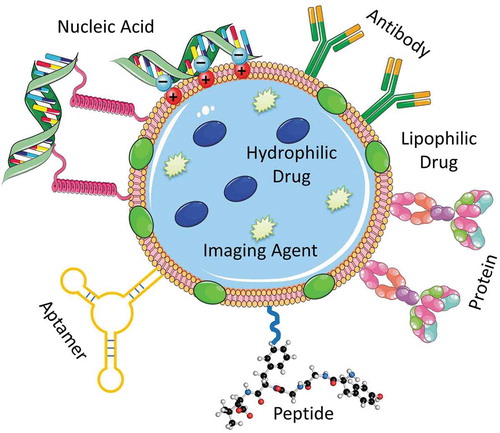
Figure 3. Mechanisms of drug transport through the blood-brain barrier using nanocarriers conjugated to receptor-specific ligands and cationized ligands. (1) Receptor-mediated endocytosis of the nanocarrier; (1a) Exocytosis of the nanocarrier; (1b) Dissociation of the receptor from the ligand-conjugated nanocarrier and acidification of the vesicle leading to the degradation of the nanocarrier and the release of the drug into the brain; (1 c and 1d) Recycling of receptors at the luminal cytoplasmic membrane; (2a) Adsorptive-mediated endocytosis of the nanocarrier conjugated to cationized ligands; (2b) Exocytosis of positively charged nanocarriers. Reproduced with permission [Citation58]
![Figure 3. Mechanisms of drug transport through the blood-brain barrier using nanocarriers conjugated to receptor-specific ligands and cationized ligands. (1) Receptor-mediated endocytosis of the nanocarrier; (1a) Exocytosis of the nanocarrier; (1b) Dissociation of the receptor from the ligand-conjugated nanocarrier and acidification of the vesicle leading to the degradation of the nanocarrier and the release of the drug into the brain; (1 c and 1d) Recycling of receptors at the luminal cytoplasmic membrane; (2a) Adsorptive-mediated endocytosis of the nanocarrier conjugated to cationized ligands; (2b) Exocytosis of positively charged nanocarriers. Reproduced with permission [Citation58]](/cms/asset/54d24d24-262d-4a38-9d47-205dc98c196a/iedd_a_1828339_f0003_oc.jpg)
Figure 4. LHRH-targeted delivery systems. (A) Examples of different architecture of nanocarriers. (B) Distribution of tritium-labeled PEG and LHRH-PEG conjugates in tumor and different organs of mice bearing xenografts of human ovarian cancer. (C) Volume of tumor in mice bearing xenografts of human ovarian cancer treated with substances indicated. Means ± SD are shown. Modified from [Citation8,Citation9,Citation16,Citation17,Citation25,Citation27,Citation46,Citation61,Citation82]
![Figure 4. LHRH-targeted delivery systems. (A) Examples of different architecture of nanocarriers. (B) Distribution of tritium-labeled PEG and LHRH-PEG conjugates in tumor and different organs of mice bearing xenografts of human ovarian cancer. (C) Volume of tumor in mice bearing xenografts of human ovarian cancer treated with substances indicated. Means ± SD are shown. Modified from [Citation8,Citation9,Citation16,Citation17,Citation25,Citation27,Citation46,Citation61,Citation82]](/cms/asset/e87976d2-2730-4940-8522-5c639277390a/iedd_a_1828339_f0004_oc.jpg)
Table 1. List of FDA-approved liposome formulations combined with drugs or biologics. Modified from [Citation75]
Table 2. Stimuli-sensitive various nanocarriers developed for drug and gene delivery application [Citation34,Citation38,Citation116,Citation117]
Figure 5. Responses of the poly(acrylic acid) (PAA) nanoparticles with different monomer to cross-linker ratios to pH and temperature. Reproduced with permission [Citation128]. The change in hydrodynamic diameter and drug release from high and low cross-linked PAA has been displayed schematically
![Figure 5. Responses of the poly(acrylic acid) (PAA) nanoparticles with different monomer to cross-linker ratios to pH and temperature. Reproduced with permission [Citation128]. The change in hydrodynamic diameter and drug release from high and low cross-linked PAA has been displayed schematically](/cms/asset/452b3867-4843-450b-a61b-61d7255fd34c/iedd_a_1828339_f0005_oc.jpg)
Figure 6. Internalization of a redox-sensitive nanocarrier, cleavage of disulfide linker and drug release inside cells. Disulfide-linked nanocarrier is stable outside the tumor microenvironment with low level of GSH. However, the disulfide bond of this nanocarrier is reduced to thiol groups after endocytosis inside the tumor cell with high level of GSH resulting in the dissociation of the nanostructure and release of the encapsulated drugs. Reproduced with permission [Citation117]
![Figure 6. Internalization of a redox-sensitive nanocarrier, cleavage of disulfide linker and drug release inside cells. Disulfide-linked nanocarrier is stable outside the tumor microenvironment with low level of GSH. However, the disulfide bond of this nanocarrier is reduced to thiol groups after endocytosis inside the tumor cell with high level of GSH resulting in the dissociation of the nanostructure and release of the encapsulated drugs. Reproduced with permission [Citation117]](/cms/asset/a30b21c9-c0f9-4605-8e66-22a7d55003e2/iedd_a_1828339_f0006_oc.jpg)
Figure 7. Enzyme-sensitive drug delivery. (A) Multifunctional liposomal nanocarrier responsive to matrix metalloproteinases (MMP2) for drug delivery via TAT-mediated internalization. mAB 2C5 – nucleosome-specific monoclonal antibody 2C5. (B) On-demand drug delivery triggered by bacterial lipase. Bacterial lipase causes cleavage of the internal PEG shell of the nanocarrier – resulting in release of the surface bioactive agents as well as encapsulated drugs. Reproduced with permission [Citation34]
![Figure 7. Enzyme-sensitive drug delivery. (A) Multifunctional liposomal nanocarrier responsive to matrix metalloproteinases (MMP2) for drug delivery via TAT-mediated internalization. mAB 2C5 – nucleosome-specific monoclonal antibody 2C5. (B) On-demand drug delivery triggered by bacterial lipase. Bacterial lipase causes cleavage of the internal PEG shell of the nanocarrier – resulting in release of the surface bioactive agents as well as encapsulated drugs. Reproduced with permission [Citation34]](/cms/asset/ea2397be-6fce-4d1a-bb29-dbba3f261736/iedd_a_1828339_f0007_oc.jpg)
Figure 8. Delivery and controlled release of doxorubicin (Dox) at cancer cells expressing hyaluronidase using mesoporous silica nanoparticles functionalized with biotin-modified hyaluronic acid (MSN-HA). (A) Drug loading steps to yield MSN-HA/Dox delivery system. Propylamine-functionalized silica (MSN-NH2) was first modified with desthiobiotin to obtain MSN-desthiobiotin, then by employing biotin (or desthiobiotin)–SA interaction, SA, and biotinylated HA were self-assembled on the external surface of MSN to yield MSN-HA. Optionally, therapeutic drug, doxorubicin, can be loaded into mesoporous silica nanoparticles to obtain MSN-HA/Dox. (B) Schematic illustration of the CD44 receptor-mediated endocytosis and triggering of drug release in tumor cells. MSN-HA/Dox is internalized by cancer cells via receptor-mediated endocytosis (HA–CD44 interaction), then loaded doxorubicin was released from the pore of MSN by the triggering of HAase and intracellular biotin. Reproduced with permission [Citation139]
![Figure 8. Delivery and controlled release of doxorubicin (Dox) at cancer cells expressing hyaluronidase using mesoporous silica nanoparticles functionalized with biotin-modified hyaluronic acid (MSN-HA). (A) Drug loading steps to yield MSN-HA/Dox delivery system. Propylamine-functionalized silica (MSN-NH2) was first modified with desthiobiotin to obtain MSN-desthiobiotin, then by employing biotin (or desthiobiotin)–SA interaction, SA, and biotinylated HA were self-assembled on the external surface of MSN to yield MSN-HA. Optionally, therapeutic drug, doxorubicin, can be loaded into mesoporous silica nanoparticles to obtain MSN-HA/Dox. (B) Schematic illustration of the CD44 receptor-mediated endocytosis and triggering of drug release in tumor cells. MSN-HA/Dox is internalized by cancer cells via receptor-mediated endocytosis (HA–CD44 interaction), then loaded doxorubicin was released from the pore of MSN by the triggering of HAase and intracellular biotin. Reproduced with permission [Citation139]](/cms/asset/59b168ec-8d0e-4f4d-a42b-de073e52e9d3/iedd_a_1828339_f0008_oc.jpg)
Figure 9. Doxorubicin (Dox) release from mesoporous silica nanoparticles functionalized with biotin-modified hyaluronic acid (MSN-HA) and antitumor effect of the formulation. (A) The biotin-and hyaluronidase-responsive release profiles of doxorubicin (Dox) under pH 6.5. The biotin- and HAase-responsive release profiles of doxorubicin (Dox) were evaluated. Drug release under pH 6.5 was conducted to mimic the condition of tumor microenvironment. Under different stimulus condition, biotin (2 mmol/L), HAase (150 U/mL), or both were added to MSN-HA/Dox solution; as a control, MSN-Dox was employed. At specified time points (1, 2, 4, 6, 12, and 24 hours), cumulative drug release was measured and compared. **P < 0.01. (B) Representative tumor images obtained from tumor-bearing mice treated for 18 days with saline (control), empty MSN-HA, free nonbound Dox, or MSN-HA/Dox. Modified with permission [Citation139]
![Figure 9. Doxorubicin (Dox) release from mesoporous silica nanoparticles functionalized with biotin-modified hyaluronic acid (MSN-HA) and antitumor effect of the formulation. (A) The biotin-and hyaluronidase-responsive release profiles of doxorubicin (Dox) under pH 6.5. The biotin- and HAase-responsive release profiles of doxorubicin (Dox) were evaluated. Drug release under pH 6.5 was conducted to mimic the condition of tumor microenvironment. Under different stimulus condition, biotin (2 mmol/L), HAase (150 U/mL), or both were added to MSN-HA/Dox solution; as a control, MSN-Dox was employed. At specified time points (1, 2, 4, 6, 12, and 24 hours), cumulative drug release was measured and compared. **P < 0.01. (B) Representative tumor images obtained from tumor-bearing mice treated for 18 days with saline (control), empty MSN-HA, free nonbound Dox, or MSN-HA/Dox. Modified with permission [Citation139]](/cms/asset/48fd6967-236e-4095-8bea-262e91a35ff7/iedd_a_1828339_f0009_oc.jpg)
Figure 10. Examples of light triggered drug delivery. (A) Schematic representation of an encapsulated in vitro transcription–translation liposomal system triggered by irradiating caged DNA with light. (B) Delivery of doxorubicin through the near-infrared-triggered induction of dehybridization of the DNA conjugated at the surface of gold nanorods. Upon irradiation of the gold nanorods with NIR laser, the resulting light to heat transformation increases local temperature and leads to the detachment of the DNA helices from the gold surface – resulting in the release of the encapsulated doxorubicin. Reproduced with permission [Citation34]
![Figure 10. Examples of light triggered drug delivery. (A) Schematic representation of an encapsulated in vitro transcription–translation liposomal system triggered by irradiating caged DNA with light. (B) Delivery of doxorubicin through the near-infrared-triggered induction of dehybridization of the DNA conjugated at the surface of gold nanorods. Upon irradiation of the gold nanorods with NIR laser, the resulting light to heat transformation increases local temperature and leads to the detachment of the DNA helices from the gold surface – resulting in the release of the encapsulated doxorubicin. Reproduced with permission [Citation34]](/cms/asset/ad0d16d3-fac5-4505-adbc-099efb089aa0/iedd_a_1828339_f0010_oc.jpg)
Figure 11. Near-infrared (NIR) light-triggered nanocarrier with reversible DNA valves for controlled release. (A) A schematic of light-controlled release of nucleic acid. Cargo loaded gold nanorods were coated with mesoporous silica shell and covered with reversible single stranded DNA valves. When such nanocarrier is irradiated with the NIR laser, the resulting photothermal effect disrupt the electrostatic bonding between silicon shell and DNA and activate the DNA valves to the ‘on’ state leading to the release of the encapsulated cargo molecules. Such release of the cargo is stopped when the NIR laser is turned off. (B) Controlled release profile of a cargo under NIR laser irradiation (808 nm, 2.7 W·cm−2) for different on/off cycles. Reproduced with permission [Citation159]
![Figure 11. Near-infrared (NIR) light-triggered nanocarrier with reversible DNA valves for controlled release. (A) A schematic of light-controlled release of nucleic acid. Cargo loaded gold nanorods were coated with mesoporous silica shell and covered with reversible single stranded DNA valves. When such nanocarrier is irradiated with the NIR laser, the resulting photothermal effect disrupt the electrostatic bonding between silicon shell and DNA and activate the DNA valves to the ‘on’ state leading to the release of the encapsulated cargo molecules. Such release of the cargo is stopped when the NIR laser is turned off. (B) Controlled release profile of a cargo under NIR laser irradiation (808 nm, 2.7 W·cm−2) for different on/off cycles. Reproduced with permission [Citation159]](/cms/asset/d9389623-7801-4d05-a681-7d93e259963a/iedd_a_1828339_f0011_oc.jpg)
Figure 12. pH-triggered release of cargo inside the tumor microenvironment (TME). pH-sensitive nanocarriers are usually made off with acid responsive polymer or moieties. At the slightly acidic pH of tumor microenvironment, the acid sensitive moieties of such nanocarriers undergo protonation leading to the disruption of the nanostructure and the release of the encapsulated therapeutics. Reproduced with permission [Citation116]
![Figure 12. pH-triggered release of cargo inside the tumor microenvironment (TME). pH-sensitive nanocarriers are usually made off with acid responsive polymer or moieties. At the slightly acidic pH of tumor microenvironment, the acid sensitive moieties of such nanocarriers undergo protonation leading to the disruption of the nanostructure and the release of the encapsulated therapeutics. Reproduced with permission [Citation116]](/cms/asset/961daf96-8805-4d4d-9db0-2864f6bd8aaa/iedd_a_1828339_f0012_oc.jpg)
Figure 13. Hypoxia-responsive drug delivery. (A) Dual-stimuli-sensitive nanoparticles prepared from polyethyleneimine-nitroimidazole micelles (PEI-NI) co-assembled with Ce6-linked hyaluronic acid (HC). Hypoxia-mediated activation was achieved by the incorporation of a hypoxia-responsive electron acceptor (nitroimidazole, NI) converted to hydrophilic 2-aminoimidazole under hypoxic conditions leading to the release of nanoparticle-loaded doxorubicin. (B) CD 44-mediated targeted delivery of doxorubicin in cancer stem cells and release of DOX in response to hypoxia generated by laser irradiation. CD44 is a cell surface receptor, which is overexpressed in most of the cancer stem cells. Hyaluronic acid (HA), a strong affinity ligand for CD 44, is usually decorated on the surface of nanoparticles for recognizing and binding to the cancer stem cell leading to the delivery of the drug to the specific tumor cell. Reproduced with permission [Citation116]
![Figure 13. Hypoxia-responsive drug delivery. (A) Dual-stimuli-sensitive nanoparticles prepared from polyethyleneimine-nitroimidazole micelles (PEI-NI) co-assembled with Ce6-linked hyaluronic acid (HC). Hypoxia-mediated activation was achieved by the incorporation of a hypoxia-responsive electron acceptor (nitroimidazole, NI) converted to hydrophilic 2-aminoimidazole under hypoxic conditions leading to the release of nanoparticle-loaded doxorubicin. (B) CD 44-mediated targeted delivery of doxorubicin in cancer stem cells and release of DOX in response to hypoxia generated by laser irradiation. CD44 is a cell surface receptor, which is overexpressed in most of the cancer stem cells. Hyaluronic acid (HA), a strong affinity ligand for CD 44, is usually decorated on the surface of nanoparticles for recognizing and binding to the cancer stem cell leading to the delivery of the drug to the specific tumor cell. Reproduced with permission [Citation116]](/cms/asset/80cfd6f0-0ff7-49c1-ac64-92fb24017ba9/iedd_a_1828339_f0013_oc.jpg)
Figure 14. Schematic representation of different stimuli-responsive multifunctional nanocarriers for targeted drug delivery applications. Various stimuli-responsive multifunctional nanocarriers such as polymeric nanoparticles, liposomes, nanostructured lipid carriers, etc. can disassemble their nanostructure under various exogenous (temperature, ultrasound, light, etc.) and endogenous (pH, enzyme, redox potential, etc.) stimuli actions resulting in release of the encapsulated drugs at the targeted disease cells
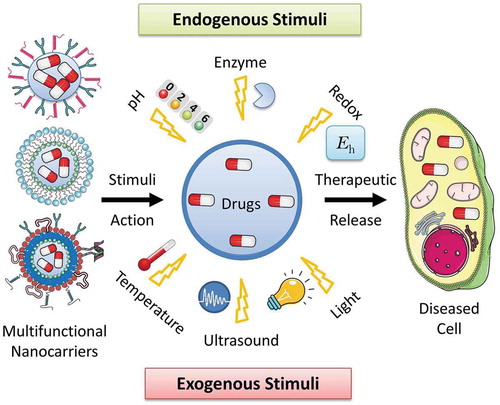
Table 3. FDA-approved nano-drug delivery systems in clinical trials. Modified from [Citation4,Citation192]