Figures & data
Figure 1. (a) Radarsat-1 InSAR image (16 August–27 October 2002) showing coseismic ground surface deformation associated with the 23 October 2002, M6.7 Nenana Mountain earthquake along the Denali Fault, Alaska, which preceded the M7.9 Denali earthquake by about 10 days. Solid line marks fault trace. (b) Modeled InSAR image using fault parameters that best fit the observed interferogram shown in (a). (c) Post-seismic deformation image and line-of-sight displacement profile showing ground response during 2003–2004 to the 3 November 2002, M7.9 Denali Fault earthquake. The image is a stack of four Radarsat-1 interferograms. Peak deformation is at a distance of ∼60 km from the fault trace (solid yellow line) and is consistent with GPS models of viscoelastic relaxation below 60 km depth.
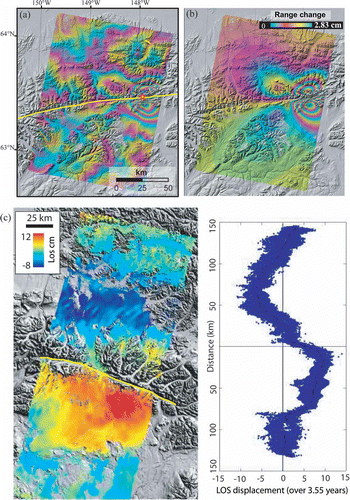
Figure 2. InSAR deformation images of selected volcanoes in the Aleutian Islands. (a) Augustine Volcano, showing deformation during 1992–1993 caused by compaction of 1986 pyroclastic flow deposits. (b) Peulik Volcano, showing as much as ∼17 cm of uplift during 1996–1997. (c) Aniakchak Volcano, showing that the caldera subsided ∼13 mm/year during 1992–2002 (averaged image). (d) Korovin Volcano, showing more than 4 cm of inflation associated with elevated seismicity from July to September 2006. (e) Okmok Volcano, showing deflation of ∼1.2 m associated with the 1997 eruption there. (f) Akutan Volcano, showing the complex deformation field that accompanied an intense earthquake swarm in March 1996 (L-band JERS-1 InSAR image). (g) Westdahl Volcano, showing inflation that occurred aseismically during 1993–1998. (h) Makushin Volcano, showing ∼7 cm of surface uplift associated with a small explosive eruption in January 1995. (i) Seguam Volcano, showing surface uplift of more than 6 cm during 1999–2000. (j) Kiska Volcano, showing subsidence due to an inferred change in the hydrothermal system during 1999–2000. All interferograms are draped over DEM-shaded relief images. Areas without interferometric coherence are uncolored. The Landsat-7 image mosaic was provided by S. Smith of the Alaska Volcano Observatory.
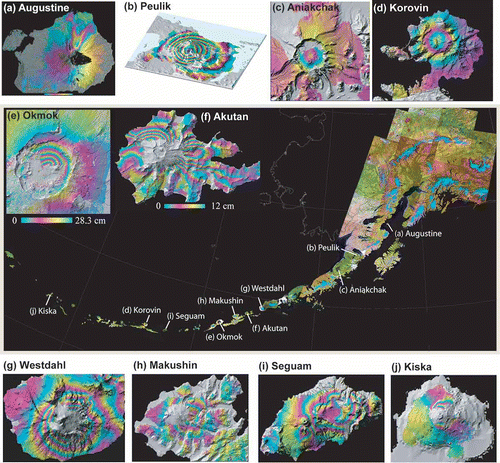
Figure 3. (a) Average deformation of Suzhou in southeastern China from L-band JERS-1 InSAR imagery. Subsidence of more than 10 cm/year during 1992–1996 is revealed by the multi-temporal InSAR images. (b) Movement of the Slumgullion landslide, Colorado, USA, mapped from a fine-beam Radarsat-1 image. Maximum displacement was more than 10 cm in 24 days during July–August 2004.
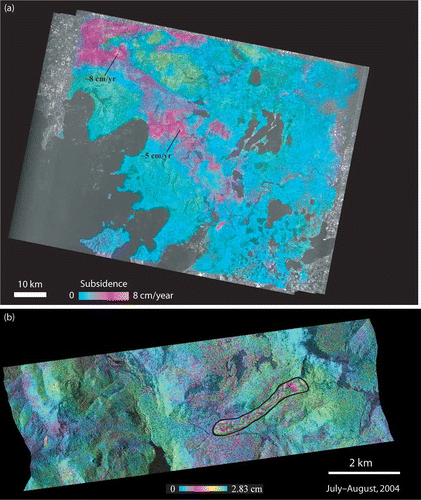
Figure 4. Movement of glaciers over Kenai Pennisula, southern Alaska in 24 hours between 12 and 13 November 1995. The interferometric phase image is draped over the radar intensity image. Each fringe (full-color cycle) represents a 2.8-cm change in range distance.
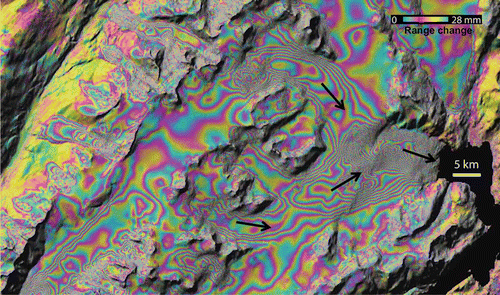
Figure 5. (a) C-band (λ = 5.7 cm) interferogram produced from Radarsat-1 images, showing heterogeneous water-level changes in swamp forests in coastal Louisiana between 22 May and 15 June 2003. The interferometric phase image is draped over the radar intensity image. Each fringe (full-color cycle) represents a 2.8 cm change in range distance or a 3.1 cm change in water level. (b) A three-dimensional view of water-level changes derived from the InSAR image.
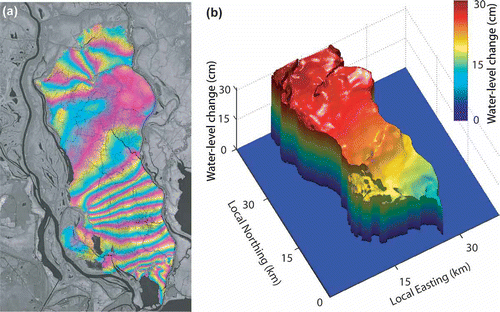
Figure 6. (a) Radarsat-1 image acquired on 2 September 2005, over New Orleans, Louisiana, USA. (b) The extent of flooding, mapped by combining the Radarsat-1 image with a pre-flood Landsat Enhanced Thematic Mapper Plus (ETM+) image mosaic. Several possible oil slicks are identified. Calm water acts as a specular reflector (or forward scatterer) of the SAR signal, resulting in very low backscatter values for flooded areas. However, flooding in areas with tall vegetation or buildings can result in very high, ‘double-bounce’ backscattering, a phenomenon that is important for identifying flooding in forests and urban areas. The tendency of oil slicks on water is to dampen the roughness of the water, which allows for discrimination of slicks in open water under moderate to light wind conditions.
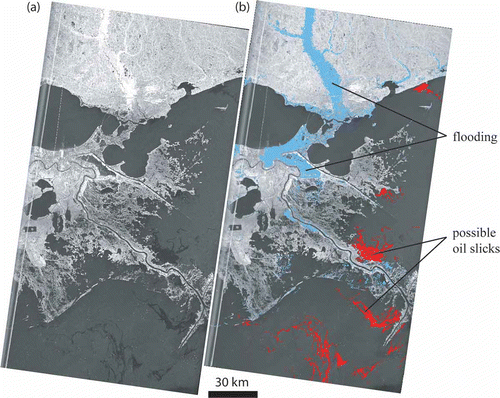
Figure 7. Thickness of lava flows emplaced during the April 1997 eruption at Okmok Volcano, Alaska. Flow thickness was derived from the height difference between pre-eruption and post-eruption DEMs that were constructed from repeat-pass InSAR images. (b) Lava thickness along profile S-S' which reached nearly 50 m in the thickest part of the flow.
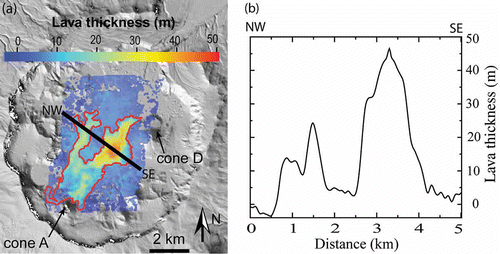
Figure 8. Averaged ERS-1/ERS-2 and Radarsat-1 intensity images of southeastern Louisiana, USA, with distinct land cover classes identified by symbols (explanation in lower left). (b) Averaged radar backscattering coefficients (relative to urban backscattering returns) for seven major land cover classes during both leaf-on and leaf-off seasons. Variations of the radar-backscattering coefficient were used to distinguish different land cover types.
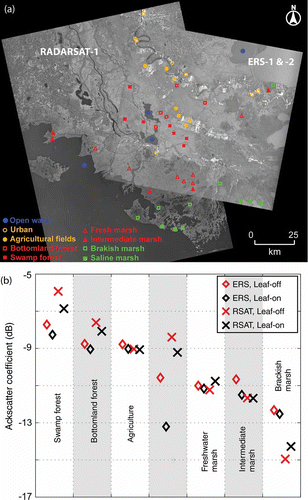
Figure 9. Radarsat-1 SAR intensity images of the Yukon River Basin, Alaska, acquired on (a) 17 August 2003, (b) 10 September 2003, and (c) 4 October 2003. These SAR images show the progression of a fire that started in July 2003 (successively larger bright areas). The derived fire severity map is shown in (d).
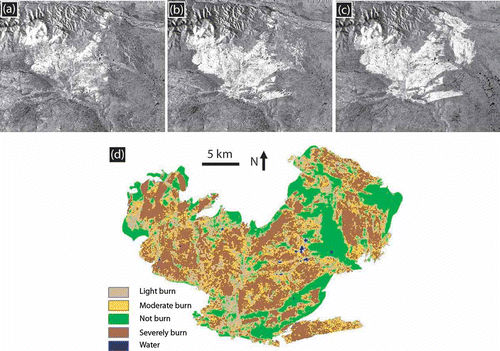