Figures & data
Figure 1. Illustration of the Eulerian and Lagrangian meshes, where and
denote the solid region and fluid region, respectively. The velocity interpolation coefficients from the locally refined mesh at the fluid-solid interface are also shown. The velocities at the new nodes are interpolated from the old nodes within the square region.
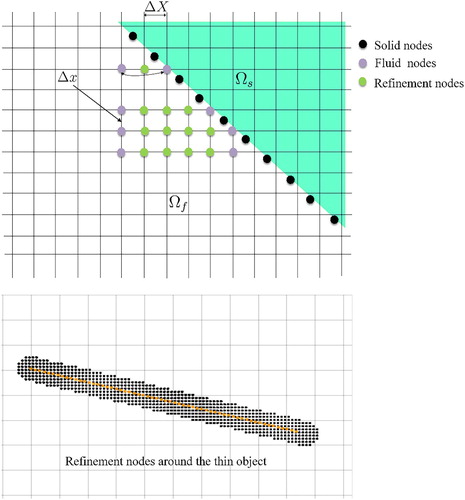
Table
Figure 2. Illustration of the background mesh and locally refined mesh within vicinity of the thin object.
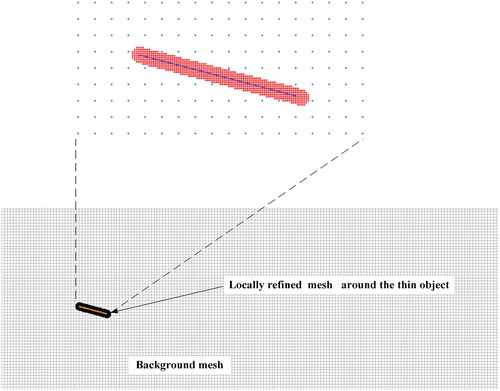
Table 1. Flow around stationary circular cylinder: 
, 
and St at 
.
Figure 4. The regression analysis for (a) Drag coefficient and (b) lift coefficient factor using AMR-IBM and CFD simulation.
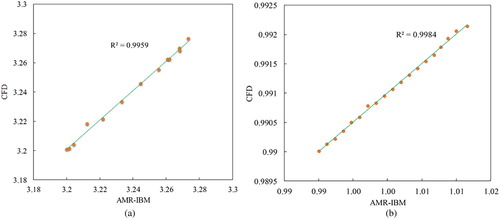
Figure 6. Variations of the (a) lift coefficient and (b) drag coefficient with time for flow around a stationary rigid thin object at obtained using the AMR-IBM algorithm. The lift and drag coefficients obtained from the IBM algorithm with full mesh refinement are plotted for comparison.
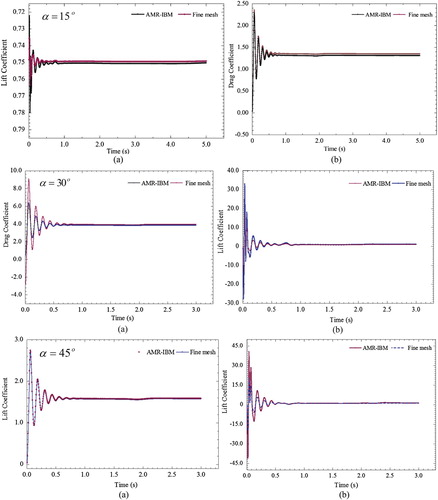
Figure 7. Velocity contour for flow around a stationary rigid thin object (a) AMR-IBM algorithm, (b) Fine mesh.
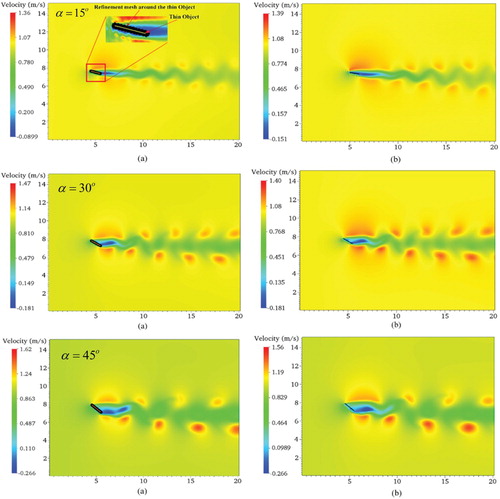
Figure 8. Velocity contour of the moving thin object inclined at an angle of attack 45° at (a) t = 1.5 s, (b) t = 3.5 s, and (c) t = 8.0 s obtained using the AMR-IBM algorithm.
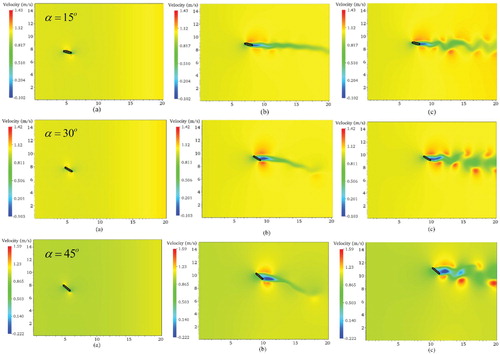
Figure 10. Snapshots of the deformed flexible filament at different time steps. Note that a fixed mesh was used for the fluid flow whereas an adaptive mesh is used within proximity of the thin elastic structure to capture the physics of fluid-solid interaction.
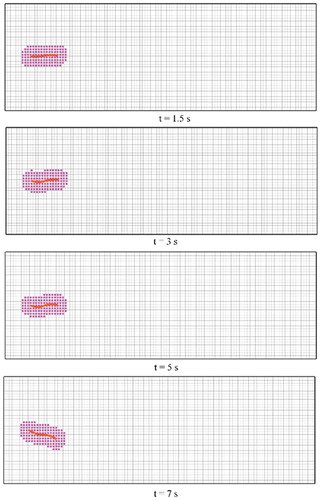
Figure 12. Two-dimensional flexible filament on velocity and geometry deformation distribution at different time step.
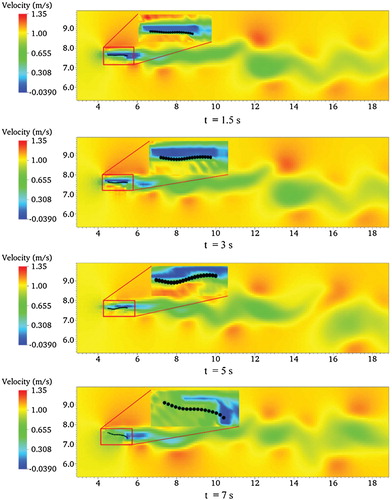