Figures & data
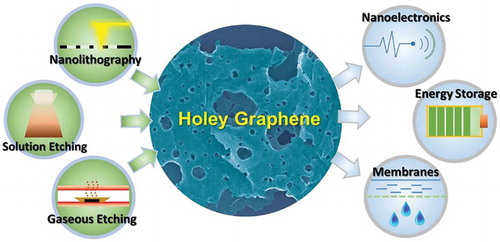
Figure 1. TEM images of a suspended graphene sheet (a) before and (b) after a nanopore is made by electron beam ablation. (c) Higher magnification image of the nanopore. (d) Multiple nanopores made in close proximity to each other. Scale bars are 50, 50, 2, and 10 nm, respectively. Reproduced from Ref. [Citation20] with permission from AIP Publishing LLC.
![Figure 1. TEM images of a suspended graphene sheet (a) before and (b) after a nanopore is made by electron beam ablation. (c) Higher magnification image of the nanopore. (d) Multiple nanopores made in close proximity to each other. Scale bars are 50, 50, 2, and 10 nm, respectively. Reproduced from Ref. [Citation20] with permission from AIP Publishing LLC.](/cms/asset/625e5bfc-1b00-4e8d-bee4-4393e1627264/tmrl_a_1271047_f0001_b.gif)
Figure 2. Schematic depiction of the fabrication of nanoperforated, highly oriented pyrolitic graphene (HOPG), or hG, using block copolymer lithography and the corresponding top-down scanning electron microscopy (SEM) images of (a) vertically oriented PMMA cylinders in a block copolymer thin film obtained by thermal annealing, (b) residual PS honeycomb template obtained after selective PMMA removal with UV irradiation, (c) etched structures after O2 followed by CHF3 + O2 plasma RIE resulting in the etching of the random copolymer mat and the oxide buffer layer, respectively, and (d) nanoperforated HOPG resulting from the final O2 plasma RIE and the removal of the oxide buffer by HF solution. Scale bars = 200 nm. Reproduced from Ref. [Citation15] with permission from American Chemical Society.
![Figure 2. Schematic depiction of the fabrication of nanoperforated, highly oriented pyrolitic graphene (HOPG), or hG, using block copolymer lithography and the corresponding top-down scanning electron microscopy (SEM) images of (a) vertically oriented PMMA cylinders in a block copolymer thin film obtained by thermal annealing, (b) residual PS honeycomb template obtained after selective PMMA removal with UV irradiation, (c) etched structures after O2 followed by CHF3 + O2 plasma RIE resulting in the etching of the random copolymer mat and the oxide buffer layer, respectively, and (d) nanoperforated HOPG resulting from the final O2 plasma RIE and the removal of the oxide buffer by HF solution. Scale bars = 200 nm. Reproduced from Ref. [Citation15] with permission from American Chemical Society.](/cms/asset/06329bef-2211-46ae-8d2b-beb136d22b86/tmrl_a_1271047_f0002_c.jpg)
Figure 3. Nanosphere lithography for hG. Reproduced from Ref. [Citation38] with permission from American Chemical Society.
![Figure 3. Nanosphere lithography for hG. Reproduced from Ref. [Citation38] with permission from American Chemical Society.](/cms/asset/8f1b7a33-cf24-4307-9fe8-609277601d23/tmrl_a_1271047_f0003_c.jpg)
Table 1. Comparison of dimensions and electronic properties of hGs fabricated by different techniques.
Figure 4. Nanosphere-templated hG growth. Reproduced from Ref. [Citation43] with permission from Nature Publishing Group.
![Figure 4. Nanosphere-templated hG growth. Reproduced from Ref. [Citation43] with permission from Nature Publishing Group.](/cms/asset/2e27c5e6-1f2b-4c2a-b08e-2f8ca80e9339/tmrl_a_1271047_f0004_c.jpg)
Figure 5. Schematic drawing (not to scale) of the introduction of in-plane pores into GO and the subsequent filtration into an HGO paper. The thermal reduction of GO sheets with in-plane porosity and removal of water molecules from the interlayers result in a three-dimensional network with interconnecting graphitic domains that retain high electrical conductivity and structural integrity and disordered porous graphene regions that provide interplane diffusion channels (rHGO). Li ions can diffuse throughout the structure rapidly through the in-plane pores and interplane channels. The interlayer spacing between graphene sheets is greatly exaggerated. The digital image shows a coin cell-size rHGO paper. Reproduced from Ref. [Citation49] with permission from American Chemical Society.
![Figure 5. Schematic drawing (not to scale) of the introduction of in-plane pores into GO and the subsequent filtration into an HGO paper. The thermal reduction of GO sheets with in-plane porosity and removal of water molecules from the interlayers result in a three-dimensional network with interconnecting graphitic domains that retain high electrical conductivity and structural integrity and disordered porous graphene regions that provide interplane diffusion channels (rHGO). Li ions can diffuse throughout the structure rapidly through the in-plane pores and interplane channels. The interlayer spacing between graphene sheets is greatly exaggerated. The digital image shows a coin cell-size rHGO paper. Reproduced from Ref. [Citation49] with permission from American Chemical Society.](/cms/asset/f5b03e7c-2605-4aa2-b529-b363a077aeaf/tmrl_a_1271047_f0005_c.jpg)
Figure 6. Preparation and structural characterization of hG foams (HGF). (a) Schematic illustration of the preparation process of HGFs and HGF films. (b) A photograph showing a free-standing HGF. (c) SEM image of interior microstructures of HGFs. Scale bar = 1 µm. (d) TEM image of hG sheets in HGFs. Scale bar = 10 nm. (e) TEM image of non-hG sheets in GFs for comparison. Scale bar = 10 nm. (f) A photograph showing HGFs before and after mechanical compression with the flexibility of the compressed HGF film shown in the inset. (g) Cross-sectional SEM image of the compressed HGF film. Scale bar = 1 µm. Reproduced from Ref. [Citation19] with permission from Nature Publishing Group.
![Figure 6. Preparation and structural characterization of hG foams (HGF). (a) Schematic illustration of the preparation process of HGFs and HGF films. (b) A photograph showing a free-standing HGF. (c) SEM image of interior microstructures of HGFs. Scale bar = 1 µm. (d) TEM image of hG sheets in HGFs. Scale bar = 10 nm. (e) TEM image of non-hG sheets in GFs for comparison. Scale bar = 10 nm. (f) A photograph showing HGFs before and after mechanical compression with the flexibility of the compressed HGF film shown in the inset. (g) Cross-sectional SEM image of the compressed HGF film. Scale bar = 1 µm. Reproduced from Ref. [Citation19] with permission from Nature Publishing Group.](/cms/asset/08f3f644-17a4-41b0-89c6-d6dc67bdf67f/tmrl_a_1271047_f0006_c.jpg)
Figure 7. (a) Schematic of the scalable synthesis of the hG sheets, (b) digital photo of the as-synthesized hG powder in bulk, and (c) Raman spectra comparison for hG obtained at 480°C/3 h and the starting graphene (t-rGO). Reproduced from Ref. [Citation18] with permission from American Chemical Society.
![Figure 7. (a) Schematic of the scalable synthesis of the hG sheets, (b) digital photo of the as-synthesized hG powder in bulk, and (c) Raman spectra comparison for hG obtained at 480°C/3 h and the starting graphene (t-rGO). Reproduced from Ref. [Citation18] with permission from American Chemical Society.](/cms/asset/be3531d7-7317-4178-a04f-f640a4bf318a/tmrl_a_1271047_f0007_c.jpg)
Figure 8. Schematic illustration for the ultra-rapid heating of GO powder to obtain and collect fluffy hG products with much increased volume. Reproduced from Ref. [Citation57] with permission from Elsevier.
![Figure 8. Schematic illustration for the ultra-rapid heating of GO powder to obtain and collect fluffy hG products with much increased volume. Reproduced from Ref. [Citation57] with permission from Elsevier.](/cms/asset/392578f4-62c2-44f2-a210-550e3f96c6d8/tmrl_a_1271047_f0008_c.jpg)
Figure 9. (A) Schematic showing the microwave exfoliation/reduction of GO and the following chemical activation of MEGO with KOH that creates pores while retaining high electrical conductivity. (B) Low magnification SEM image of a three-dimensional a-MEGO piece. (C) High-resolution SEM image of a different sample region that demonstrates the porous morphology. (D) Annular dark-field scanning transmission electron microscopy image of the same area as (C), acquired simultaneously. As seen, a-MEGO contains micro- and mesopores with a distribution of sizes between ∼1 and ∼10 nm. (E) High-resolution phase contrast electron micrograph of the thin edge of an a-MEGO chunk, taken at 80 kV. There is a variation in focus across the image because of the sloped nature of the sample and changes in sample thickness. The image shows the presence of a dense network of nanometer scale pores surrounded by highly curved, predominantly single-layer carbon. (F) Exit wave reconstructed high-resolution transmission electron microscopy image from the edge of a-MEGO. The in-plane carbon atoms are clearly resolved, and a variety of n-membered carbon rings can be seen. Substantial curvature of the single-carbon sheets is visible, with the in-plane crystallinity being preserved. Reproduced from Ref. [Citation64] with permission from The American Association for the Advancement of Science.
![Figure 9. (A) Schematic showing the microwave exfoliation/reduction of GO and the following chemical activation of MEGO with KOH that creates pores while retaining high electrical conductivity. (B) Low magnification SEM image of a three-dimensional a-MEGO piece. (C) High-resolution SEM image of a different sample region that demonstrates the porous morphology. (D) Annular dark-field scanning transmission electron microscopy image of the same area as (C), acquired simultaneously. As seen, a-MEGO contains micro- and mesopores with a distribution of sizes between ∼1 and ∼10 nm. (E) High-resolution phase contrast electron micrograph of the thin edge of an a-MEGO chunk, taken at 80 kV. There is a variation in focus across the image because of the sloped nature of the sample and changes in sample thickness. The image shows the presence of a dense network of nanometer scale pores surrounded by highly curved, predominantly single-layer carbon. (F) Exit wave reconstructed high-resolution transmission electron microscopy image from the edge of a-MEGO. The in-plane carbon atoms are clearly resolved, and a variety of n-membered carbon rings can be seen. Substantial curvature of the single-carbon sheets is visible, with the in-plane crystallinity being preserved. Reproduced from Ref. [Citation64] with permission from The American Association for the Advancement of Science.](/cms/asset/546c8140-a61d-48e9-8010-d5a6aca87538/tmrl_a_1271047_f0009_c.jpg)
Figure 10. A typical catalytic oxidative etching process to prepare hG. Reproduced from Ref. [Citation72] with permission from The Royal Society of Chemistry.
![Figure 10. A typical catalytic oxidative etching process to prepare hG. Reproduced from Ref. [Citation72] with permission from The Royal Society of Chemistry.](/cms/asset/e5dff1ec-fb05-4c91-ba60-a8835ae04211/tmrl_a_1271047_f0010_c.jpg)
Figure 11. Several representative electron microscopy images of an Ag-graphene sample subjected to air oxidation at 300°C for 3 h: (a) a lower magnification SEM image showing both holes and tracks, SEM images showing areas enriched with (b) lower aspect ratio holes and (c) high-aspect ratio holes (ie tracks), (d) a TEM image at higher magnification showing the morphology of a hole. Reproduced from Ref. [Citation72] with permission from The Royal Society of Chemistry.
![Figure 11. Several representative electron microscopy images of an Ag-graphene sample subjected to air oxidation at 300°C for 3 h: (a) a lower magnification SEM image showing both holes and tracks, SEM images showing areas enriched with (b) lower aspect ratio holes and (c) high-aspect ratio holes (ie tracks), (d) a TEM image at higher magnification showing the morphology of a hole. Reproduced from Ref. [Citation72] with permission from The Royal Society of Chemistry.](/cms/asset/fac6e01b-9d30-4c23-9509-61db0c49964a/tmrl_a_1271047_f0011_b.gif)
Figure 12. Proposed mechanism for the photoinduced reaction between RGO (ie rGO), AuNPs (ie Au nanoparticles), and H2O2. A suspension of RGO, AuNPs, and H2O2 is prepared as shown at t = 0. Upon irradiation, AuNPs catalyze photolytic production of OH• as well as OH• attack on RGO. Competitive decomposition of H2O2 to O2 leads to bubble nucleation and detachment from the RGO surface, to which we attribute the observed wrinkling of the RGO sheets. Extended irradiation leads to migration of AuNPs and creation of nanopores at various places along the RGO sheets through diffusion and/or adsorption/desorption. Reproduced from Ref. [Citation53] with permission from American Chemical Society.
![Figure 12. Proposed mechanism for the photoinduced reaction between RGO (ie rGO), AuNPs (ie Au nanoparticles), and H2O2. A suspension of RGO, AuNPs, and H2O2 is prepared as shown at t = 0. Upon irradiation, AuNPs catalyze photolytic production of OH• as well as OH• attack on RGO. Competitive decomposition of H2O2 to O2 leads to bubble nucleation and detachment from the RGO surface, to which we attribute the observed wrinkling of the RGO sheets. Extended irradiation leads to migration of AuNPs and creation of nanopores at various places along the RGO sheets through diffusion and/or adsorption/desorption. Reproduced from Ref. [Citation53] with permission from American Chemical Society.](/cms/asset/78da78f4-18b9-457d-874e-068137465560/tmrl_a_1271047_f0012_c.jpg)
Figure 13. The fabrication of N-doped hG foam (N-GMF). (a) Pyrrole (Py) monomer dispersed in a GO suspension. (b) Three-dimensional polypyrrole/graphene (Py/G) obtained after hydrothermal treatment. (c) N-GMF with intercalated Fe2O3 nanoparticles. (d) N-GMF after being washed with HCl. Reproduced from Ref. [Citation84] with permission from The Royal Society of Chemistry.
![Figure 13. The fabrication of N-doped hG foam (N-GMF). (a) Pyrrole (Py) monomer dispersed in a GO suspension. (b) Three-dimensional polypyrrole/graphene (Py/G) obtained after hydrothermal treatment. (c) N-GMF with intercalated Fe2O3 nanoparticles. (d) N-GMF after being washed with HCl. Reproduced from Ref. [Citation84] with permission from The Royal Society of Chemistry.](/cms/asset/8ae4178f-2fec-4340-b322-eef3bbcdb02e/tmrl_a_1271047_f0013_c.jpg)
Figure 14. (a) Digital photos of h-Graphene (ie hG) and graphene filtration films. The graphene film cracked during the drying process. (b) Mechanical tensile test result for the h-Graphene film. (c) Cross-sectional SEM images for h-Graphene (left) and graphene films (right) at equivalent areal mass densities of 0.31 mg/cm2. Cross-sectional SEM images at identical magnifications for h-Graphene (d) and graphene films (e). Reproduced from Ref. [Citation18] with permission from American Chemical Society.
![Figure 14. (a) Digital photos of h-Graphene (ie hG) and graphene filtration films. The graphene film cracked during the drying process. (b) Mechanical tensile test result for the h-Graphene film. (c) Cross-sectional SEM images for h-Graphene (left) and graphene films (right) at equivalent areal mass densities of 0.31 mg/cm2. Cross-sectional SEM images at identical magnifications for h-Graphene (d) and graphene films (e). Reproduced from Ref. [Citation18] with permission from American Chemical Society.](/cms/asset/c7bff96a-4000-4bdd-b8d4-b262d3d3453b/tmrl_a_1271047_f0014_c.jpg)
Figure 15. Preparation and electrochemical characterizations of hG paper (HGP) and graphene paper (GP)-based supercapacitors in 1.0 M H2SO4 aqueous electrolyte. (a) Photographs of aqueous dispersions of hGO and hG. (b) Photograph of a free-standing flexible HGP with a thickness of ∼9 µm. (c) SEM image of the cross-section of the HGP. (d) Schematic illustration of ion diffusion pathways across the GP and HGP. (e) Cyclic voltammetry (CV) curves at a scan rate of 200 mV/s. Galvanostatic charge/discharge curves at a current density of 1 A/g (f) and 20 A/g (g). (h) Specific capacitances vs. current densities. (i) Nyquist plots. Reproduced from Ref. [Citation52] with permission from American Chemical Society.
![Figure 15. Preparation and electrochemical characterizations of hG paper (HGP) and graphene paper (GP)-based supercapacitors in 1.0 M H2SO4 aqueous electrolyte. (a) Photographs of aqueous dispersions of hGO and hG. (b) Photograph of a free-standing flexible HGP with a thickness of ∼9 µm. (c) SEM image of the cross-section of the HGP. (d) Schematic illustration of ion diffusion pathways across the GP and HGP. (e) Cyclic voltammetry (CV) curves at a scan rate of 200 mV/s. Galvanostatic charge/discharge curves at a current density of 1 A/g (f) and 20 A/g (g). (h) Specific capacitances vs. current densities. (i) Nyquist plots. Reproduced from Ref. [Citation52] with permission from American Chemical Society.](/cms/asset/b9f13fc2-f237-4c5a-aa1d-97822babe9c6/tmrl_a_1271047_f0015_c.jpg)
Figure 16. Electrochemical characterization of ∼5 µm thick Si–hG paper anodes. (a) Specific delithiation capacity (solid) and coulombic efficiency (open) of Si–hG between 0.02 and 1.5 V at 1 and 8 A/g (C/3 and 2.6 C based on a theoretical capacity of 3052 mAh/g), and between 0.1 and 0.55 V at 4 A/g (1.3 C). (b) Fifth-cycle charge/discharge curves of Si–hG at 1, 4, and 8 A/g. Reproduced from Ref. [Citation17] with permission from John Wiley and Sons. A C-rate of 1C is defined to provide the full battery capacity at a 1-h discharge; 0.5C or C/2 is a 2-h discharge and 0.2C or C/5 is a 5-h discharge [Citation92].
![Figure 16. Electrochemical characterization of ∼5 µm thick Si–hG paper anodes. (a) Specific delithiation capacity (solid) and coulombic efficiency (open) of Si–hG between 0.02 and 1.5 V at 1 and 8 A/g (C/3 and 2.6 C based on a theoretical capacity of 3052 mAh/g), and between 0.1 and 0.55 V at 4 A/g (1.3 C). (b) Fifth-cycle charge/discharge curves of Si–hG at 1, 4, and 8 A/g. Reproduced from Ref. [Citation17] with permission from John Wiley and Sons. A C-rate of 1C is defined to provide the full battery capacity at a 1-h discharge; 0.5C or C/2 is a 2-h discharge and 0.2C or C/5 is a 5-h discharge [Citation92].](/cms/asset/5318da1d-9d70-48fb-bbb9-5594c23097d6/tmrl_a_1271047_f0016_b.gif)
Figure 17. (a) Rotating disk electrode voltammograms of the reduced graphene, reduced holey graphene, nitrogen-doped holey graphene (NHG), and 20 wt% Pt–C electrodes in an O2-saturated, 0.1 M KOH solution at a rotation rate of 1600 rpm. Scan rate: 10 mV/s; (b) rotating ring-disk electrode voltammogram of the NHG electrode in an O2-saturated, 0.1 M KOH aqueous solution at a scan rate of 10 mV/s at 1600 rpm; (c) current–time chronoamperometric response for ORR at the NHG electrode and the 20 wt% Pt–C electrode upon introduction of 1 M methanol after about 300 s at −0.25 V; (d) chronoamperometric response of the NHG electrode and the 20 wt% Pt–C electrode at −0.25 V in an O2-saturated 0.1 M KOH aqueous solution. Reproduced from Ref. [Citation96] with permission from The Royal Society of Chemistry.
![Figure 17. (a) Rotating disk electrode voltammograms of the reduced graphene, reduced holey graphene, nitrogen-doped holey graphene (NHG), and 20 wt% Pt–C electrodes in an O2-saturated, 0.1 M KOH solution at a rotation rate of 1600 rpm. Scan rate: 10 mV/s; (b) rotating ring-disk electrode voltammogram of the NHG electrode in an O2-saturated, 0.1 M KOH aqueous solution at a scan rate of 10 mV/s at 1600 rpm; (c) current–time chronoamperometric response for ORR at the NHG electrode and the 20 wt% Pt–C electrode upon introduction of 1 M methanol after about 300 s at −0.25 V; (d) chronoamperometric response of the NHG electrode and the 20 wt% Pt–C electrode at −0.25 V in an O2-saturated 0.1 M KOH aqueous solution. Reproduced from Ref. [Citation96] with permission from The Royal Society of Chemistry.](/cms/asset/706e1960-ef91-4399-999d-ed2d3ee2617c/tmrl_a_1271047_f0017_c.jpg)
Figure 18. LIB performance of neat graphene (G), hG, n-doped graphene (N-G), and N-doped hG (N-hG) measured in the voltage range of 0.02–2.5 V. (a) Initial discharge–charge curves at 0.1 A/g, (b) rate capability, (c) discharge–charge curves of N-hG at various current densities, (d) capacity retention vs. various current densities, and (e) cycling performance at 5 A/g. Reproduced from Ref. [Citation95] with permission from John Wiley and Sons.
![Figure 18. LIB performance of neat graphene (G), hG, n-doped graphene (N-G), and N-doped hG (N-hG) measured in the voltage range of 0.02–2.5 V. (a) Initial discharge–charge curves at 0.1 A/g, (b) rate capability, (c) discharge–charge curves of N-hG at various current densities, (d) capacity retention vs. various current densities, and (e) cycling performance at 5 A/g. Reproduced from Ref. [Citation95] with permission from John Wiley and Sons.](/cms/asset/3a782618-e6ec-4856-b7d6-83086246651a/tmrl_a_1271047_f0018_b.gif)
Figure 19. Four new concepts using graphene nanostructures for DNA sequencing. (a) Detection of changes in the ionic current through a nanopore in a graphene membrane due to the passage of a DNA molecule. (b) Modulations of a tunneling current through a nanogap between two graphene electrodes due to the presence of a DNA molecule. (c) Variations in the in-plane current through a graphene nanoribbon due to traversal of a DNA molecule. (d) Changes in a graphene current due to the physisorption of DNA bases onto the graphene. Reproduced from Ref. [Citation106] with permission from Nature Publishing Group.
![Figure 19. Four new concepts using graphene nanostructures for DNA sequencing. (a) Detection of changes in the ionic current through a nanopore in a graphene membrane due to the passage of a DNA molecule. (b) Modulations of a tunneling current through a nanogap between two graphene electrodes due to the presence of a DNA molecule. (c) Variations in the in-plane current through a graphene nanoribbon due to traversal of a DNA molecule. (d) Changes in a graphene current due to the physisorption of DNA bases onto the graphene. Reproduced from Ref. [Citation106] with permission from Nature Publishing Group.](/cms/asset/d1bccc90-9d27-4486-8b6e-5229f093abe3/tmrl_a_1271047_f0019_c.jpg)
Table 2. Comparison of ion-exchange capacity (measured at 65°C), proton conductivity (measured at 65°C), and methanol permeability for the various GO/hGO membranes in comparison to the benchmark Nafion® 112 membrane.
Figure 20. (a) CG model for the patterned hG. (b)–(g) Snapshots of the deformed hG under various applied tensile strains. The arrow in (b) indicates the direction of applied tensile load. (h) Resultant force in the hG as a function of applied elongation. The light green shaded region (‘Stage I’) highlights the extremely compliant region of the hG while the light blue shaded region (‘Stage II’) highlights the highly compliant region with an effective stiffness of about 15.8 N/m. Here, LG = 351 nm, WG = 199 nm, LC = 50 nm, and WR = 2 nm. Reproduced from Ref. [Citation114] with permission from AIP Publishing LLC.
![Figure 20. (a) CG model for the patterned hG. (b)–(g) Snapshots of the deformed hG under various applied tensile strains. The arrow in (b) indicates the direction of applied tensile load. (h) Resultant force in the hG as a function of applied elongation. The light green shaded region (‘Stage I’) highlights the extremely compliant region of the hG while the light blue shaded region (‘Stage II’) highlights the highly compliant region with an effective stiffness of about 15.8 N/m. Here, LG = 351 nm, WG = 199 nm, LC = 50 nm, and WR = 2 nm. Reproduced from Ref. [Citation114] with permission from AIP Publishing LLC.](/cms/asset/e353a303-10a6-4bc0-a3e8-a25c5b1525ff/tmrl_a_1271047_f0020_b.gif)