Figures & data
Figure 1 The enhanced permeability and retention effect operating in tumor milieu permitting accumulation of nanometer-sized particles in cancer cells. Blood vessels in tumor tissue have defective architecture with gaps as large as 200–1000 nm allowing nanoparticles to extravasate and accumulate inside the tumor tissue. The retention time of drugs packed in nanoparticles is ten times higher than that of unpacked drugs, which eventually return to the vascular system. This phenomenon of permeability of molecules due to their size is a boon to cancer therapy and is known as the enhanced permeability and retention effect.
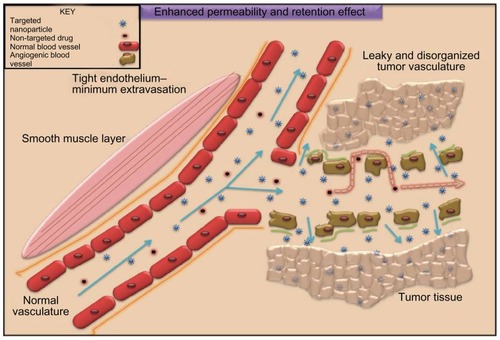
Figure 2 Passive targeting of nanoparticles to tumor cells according to tumor vasculature and size characteristics. Passive targeting of nanoparticles: nanoparticles (in yellow) concentrate to tumor sites taking advantage of leaky vasculature and diminished lymphatics. Tumor angiogenesis is torturous and aberrant with gap sizes of 100 nm–2 μm. Nanocarriers because of their small size can thus accumulate in tumor interstitium minimizing systemic toxicity and enhancing tumor cell killing. Free or uncoated anticancer drugs (blue) lack this advantage accounting for serious side effects due to extravasation to healthy cells and reflux at target sites. Yellow arrows indicate the extravasation of nanocarriers to the tumor site (indicated with thickened arrows, in addition, to represent higher biodistribution). Blue arrows indicate the extravasation of the uncoated drug to the nontargeting site rendering systemic toxicity, which is further thickened to symbolize higher concentration of the uncoated drug.
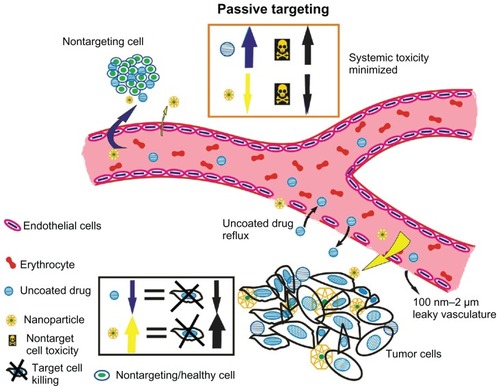
Figure 3 Passive targeting of nanoparticles to tumor cells with stealth characteristics to evade rapid destruction by reticuloendothelial system and excretion. Passive targeting of PEGylated nanoparticles. Nanoparticles surface coated with poly(ethylene glycol) (PEG) are capable of evading reticuloendothelial system (RES), thus delaying elimination to liver and spleen. Nanoparticles uncoated with PEG are easily recognized by the immune cells (phagocytes and monocytes) resulting in earlier elimination to liver and spleen. This results in decreased blood circulation and enhanced tumor localization of the anticancer nanovector.
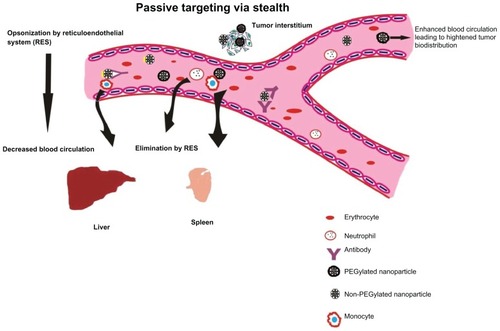
Table 1 Clinically approved nanoparticle systems and their indication
Figure 4 Active targeting of nanoparticles to tumor cells according to the presence of tumor-seeking addresses on nanoparticle surface promoting tumor residence. Active nanoparticle targeting: the nanoparticle is surface tagged with a certain ligand against a tumor-specific antigen. This enables direct targeting to target site. The model here discusses dual targeting effected in a synergistic fashion: targeting endothelial cell receptors (vascular cellular adhesion molecules, vascular endothelial growth factor, etc) such as angiogenesis antagonists and monoclonal antibodies against typical tumor signatures such as endothelial growth factor receptors, HER2, which result in tumor cell apoptosis. Endothelial cell targeting curbs angiogenesis of tumor interstitium preventing oxygen supply and nutrients while tumor targeting causes direct cell killing as the nanoparticle encapsulating the anticancer drug is taken up by the cell. (1) The nanoparticle is targeted against endothelial cell receptor and against a tumor antigen. (2) The nanoparticle is taken up by the tumor cell via endocytosis wherein nanocarrier vesicles formed release the contents in a pH-dependent manner. The anticancer drug(s) embedded in the nanovector is(are) released and translocated to the nucleus. (3) Action of anticancer drug results in DNA fragmentation that finally leads to (4) programmed cell death.
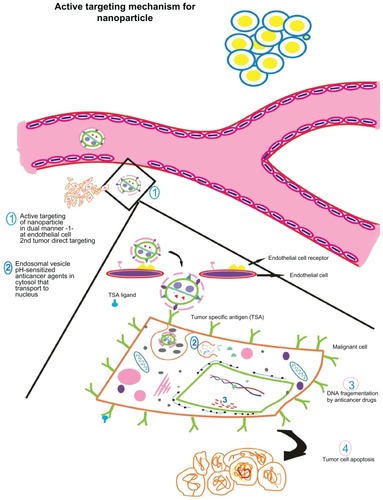
Table 2 Important nanovector paradigms against typical tumor signatures
Figure 5 A model for multifunctional nanoparticle with desired characteristics possessing multiple drugs, dual tumor-targeting ligands, cell-penetrating peptide, and imaging agents.
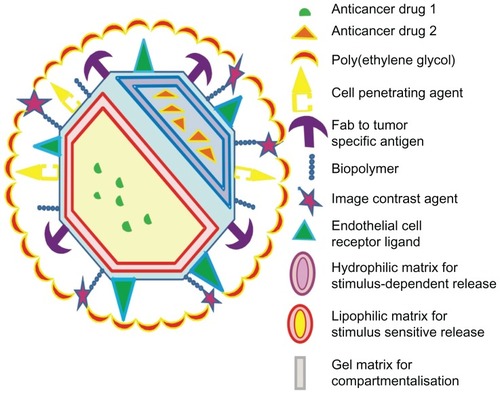
Figure 6 Schematic representation of timelines depicting the clinical approval of nanoparticle-based drug vehicles for human diseases.
