Figures & data
Figure 1 The pathophysiology of OA. OA is a complex disease with multi-factors and causes, which affects not only the cartilage tissue but also the surrounding tissues. (A) Pathological knee. (B) OA not only impacts cartilage but also exerts its effects on the surrounding tissues. This results in the modulation of the subchondral plate, synovial tissue inflammation, and the development of erosion and osteophytes in the supporting bone. (C) The primary etiological event responsible for OA remains largely elusive. Several risk factors have been proposed to augment susceptibility to OA, including aging, chronic mechanical overloading, traumatic injury, genetic predisposition, sex, and hormonal variations. (D) Numerous regulatory pathways contribute to the initiation and progression of OA, although not all are necessarily involved in every disease phenotype. (E) Cells within the affected tissues actively participate in the initiation and propagation of OA. (F) During the course of OA, these cells undergo phenotypic changes, leading to altered transcriptome profiles that perpetuate an unending cycle of inflammation and tissue deterioration. Reproduced with permission from Foo JB, Looi QH, How CW, et al. Mesenchymal Stem Cell-Derived Exosomes and MicroRNAs in Cartilage Regeneration: biogenesis, Efficacy, miRNA Enrichment and Delivery. Pharmaceuticals. 2021;14(11):56.Citation13 Copyright 2021, MDPI. Creative Commons.
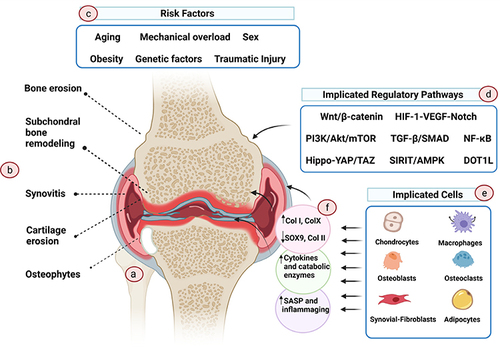
Figure 2 Schematic illustration of bioprinting scaffold fabrication and scaffold treatment for osteochondral defects in OA. (A) The fabrication of 3D-bioprinted MSC-laden biomimetic multiphasic scaffolds. (B) The designed scaffold was implanted into a severe joint injury rat model with osteochondral defects for evaluation. Reprinted from Biomaterials, 279, Liu Y, Peng L, Li L, et al 3D-bioprinted BMSC-laden biomimetic multiphasic scaffolds for efficient repair of osteochondral defects in an osteoarthritic rat model. 121216. Copyright 2021, with permission from Elsevier.Citation25
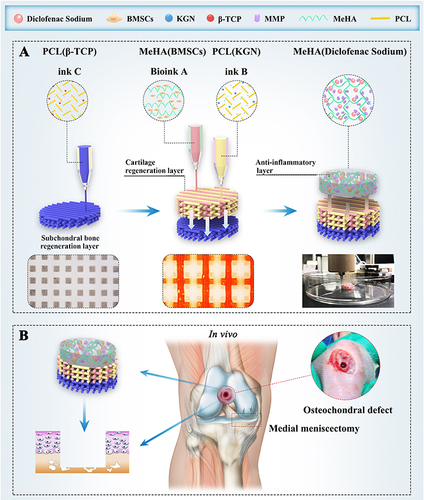
Figure 3 Schematic illustration of the main content of inkjet bioprinting. (A) Concise depiction of the cellular environment reveals the presence of cell-fate associated biomolecules that are secreted by cells, existing in both liquid and solid phases within the living organism. (B) High resolution and overprinting strategy developed inkjet bioprinting. (C) Concentration gradient, pattern, and molecular pattern alone or their combination can be printed by the inkjet printer. (D) Through the application of printing technology, biomolecule patterns have been utilized in the study of cellular behavior and tissue regeneration. Reproduced with permission from Li X, Liu B, Pei B, et al. Inkjet Bioprinting of Biomaterials. Chem Rev. 2020;120(19):10793–10833.Citation49 Copyright 2020, American Chemical Society.
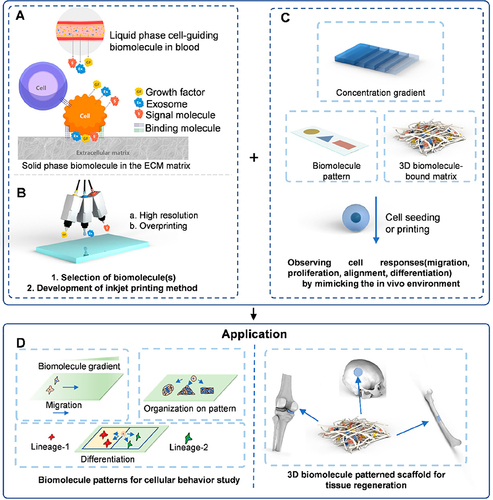
Figure 4 Schematic representation of the GelHACS-MA bioink design and the 3D bioprinting process. (A) Gelatin was functionalized by attaching amino groups with methacrylates, resulting in the formation of Gel-MA. The red text “RGD” signifies the incorporation of arginine-glycine-aspartic acid (RGD) into the GelHACS-MA bioink preparation. (B) Hyaluronic acid underwent functionalization by attaching methacrylates to its hydroxyl groups, yielding HA-MA. (C) Chondroitin sulfate was also modified by methacrylate incorporation into its hydroxymethyl groups, resulting in CS-MA. (D) GelHACS-MA hydrogels were subsequently formed, wherein Gel-MA, HA-MA, and CS-MA prepolymers were covalently cross-linked in the presence of the LAP photoinitiator, facilitated by blue light exposure, to establish a stable hydrogel network. (E) Isolation and extraction of synovial membrane-derived mesenchymal stem cells (SMSCs). The red text “Synovium” highlights the primary pathological changes in the synovium of the OA joint. (F) The outline of the 3D bioprinting strategy employing GelHACS-MA bioink. Reproduced with permission from Sang S, Mao X, Cao Y, et al 3D Bioprinting Using Synovium-Derived MSC-Laden Photo-Cross-Linked ECM Bioink for Cartilage Regeneration. ACS Appl Mater Interfaces. 2023.Citation74 Copyright 2023, American Chemical Society.
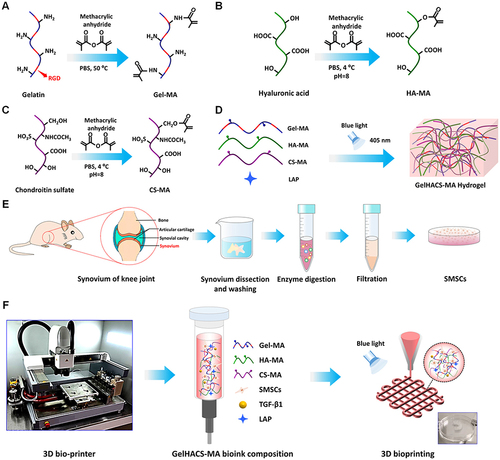
Figure 5 Schematic representation of the one-step DDS designed to facilitate osteochondral defect regeneration. The DDS involves stereolithography-based bioprinting of ECM/gelatin methacryloyl/exosome construct, followed by implantation into the osteochondral defect. (A) Utilizing stereolithography, an ECM combined with Gelatin Methacryloyl and exosome-based bioprinting is employed, followed by the implantation of the construct into the osteochondral defect. (B) Chondrocytes are observed migrating to the regions of the defect. (C) The controlled administration of exosomes is achieved through the use of 3D printed scaffolds. (D) The scaffolds lead to enhanced mitochondrial biogenesis in chondrocytes. Reproduced under the terms of a Creative Commons Attribution 4.0 International License from Chen P, Zheng L, Wang Y, et al. Desktop-stereolithography 3D printing of a radially oriented extracellular matrix/mesenchymal stem cell exosome bioink for osteochondral defect regeneration. Theranostics. 2019;9(9):2439–2459.Citation78 Copyright 2019, The Authors.
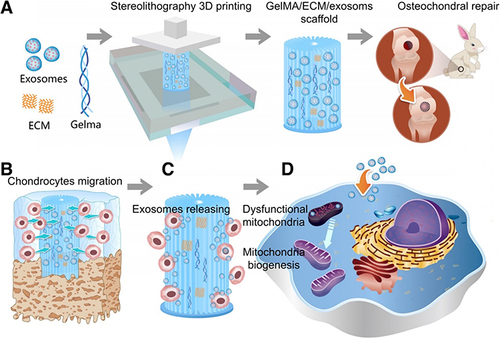
Figure 6 An example for bioprinting cell therapy for Cartilage Repair. (A) 3D-bioprinted cartilage scaffold for implantation. (a) Schematic representation of the fabricated scaffolds for cartilage repair in rabbit knee. (b) 3D computer-aided design (CAD) renderings of individual layers of the cartilage scaffold. (c-d) Dispensing path (outlined in yellow box in b) for the aligned PCL (Polycaprolactone) and hydrogel (outlined in green box in c). The GDF5-conjugated BMSC-laden hydrogel was dispensed into the space between PCL fibers. (e) Macroscopic view of GDF5-conjugated BMSC-laden hydrogel printed using OPUS. (f) Microscopic observation of the hydrogel under light microscopy. (g) Macroscopic view of the cartilage scaffold incorporating GDF5-conjugated BMSC-laden hydrogel and PCL as the supporting structure. (h) Scanning electron microscopy (SEM) images of GDF5-conjugated PLGA μS (Poly(lactic-co-glycolic acid) microspheres). (i) Implantation process of the cartilage scaffold into the defect site in a rabbit knee. (j) High-resolution image of the implanted scaffold shown in (i). (k) Microscopic visualization of the alignment of PCL and hydrogel within the scaffold. (l) High-resolution image of the area outlined in the blue box in (k). (m-p) Evaluation of minimal toxicity and distribution of PLGA μS within the BMSC-laden hydrogel in the scaffolds. (B) Mechanical properties of different components and the cross-linked hydrogel measured at 17°C. (C) Dynamic thermal rheological observations of the cross-linkage of GFHG. (D) Degradation rate of BMSC-laden hydrogel. E. Degradation rate of PCL in vitro and in vivo. Reproduced under the terms of a Creative Commons Attribution 4.0 International License from Sun Y, You Y, Jiang W, Zhai Z, Dai K. 3D-bioprinting a genetically inspired cartilage scaffold with GDF5-conjugated BMSC-laden hydrogel and polymer for cartilage repair. Theranostics. 2019;9(23):6949–6961.Citation84 Copyright 2019, The Authors.
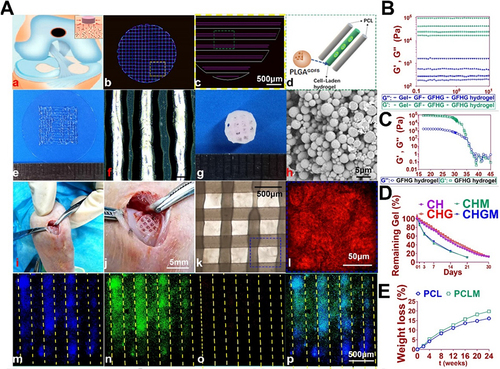