Figures & data
Figure 1. PDA phenotypes revealed in ERG recordings of nina and ina mutants and wild type. The stimulus protocol is shown at the top: three bright blue stimuli (filled rectangles) each of 4 s duration presented at 20-s intervals followed by three bright orange stimuli (unfilled rectangles) also of 4 s duration presented at 20-s intervals. The first blue stimulus generates a large response that lasts the duration of the stimulus (light-coincident component) in flies of all genotypes (unfilled arrowheads). In wild type, the PDA is generated at the termination of the blue stimulus and maintained throughout the two subsequent blue stimuli (Trace A). No PDA is generated in ninaDP245, ninaEP332, or inaEN125 (Traces B, C, and E), and partial PDAs are generated in ninaCP238 and inaCP209 (Traces D and F). During the fully developed PDA in wild type, the R1–6 photoreceptors are inactivated, and only small responses originating from R7/8 photoreceptor are elicited by the second and third blue stimuli (Trace A, filled arrowheads; also see inset). R1–6 photoreceptors of inaEN125 and inaCP209 are also inactivated by the first blue stimulus and generate only small responses to the second and third blue stimuli (Traces E and F, filled arrowheads). Thus, in these ina mutants, the afterpotential (PDA) is not present, but the R1–6 photoreceptors are inactivated, hence the name inactivation but no afterpotential. In strong nina mutants, ninaDP245 and ninaEP332, the PDA is not present and the R1–6 photoreceptors are not inactivated, generating full-amplitude responses to the second and third blue stimuli (Traces B and C, filled arrowheads). Therefore these mutants were named neither inactivation nor afterpotential. The mutant ninaCP238 displays a partial PDA and modest inactivation of R1–6 photoreceptors. The inset illustrates the R7/8 origin of the small responses to the second and third blue stimuli in wild type (Trace A, filled arrowheads). It compares the ERG of wild type (bottom) with that of the transgenic fly (top) carrying wild-type norpA cDNA driven by Rh1 promotor on a norpAP24 mutant background (norpAP24; Rh1-norpA+). The stimulus protocol is shown at the bottom. Since norpAP24 blocks phototransduction and Rh1 drives the expression of wild-type norpA cDNA only in R1–6 cells, phototransduction is blocked in R7/8 cells but the block is rescued in R1–6 cells in this transgenic fly. Note that the small response to the second blue stimulus superposed on the PDA is not present in the transgenic fly. This figure was originally published in the Journal of Biological Chemistry: Pearn, Randall, Shortridge, Burg, & Pak, 1996, J Biol Chem, 271, 4937–4945.
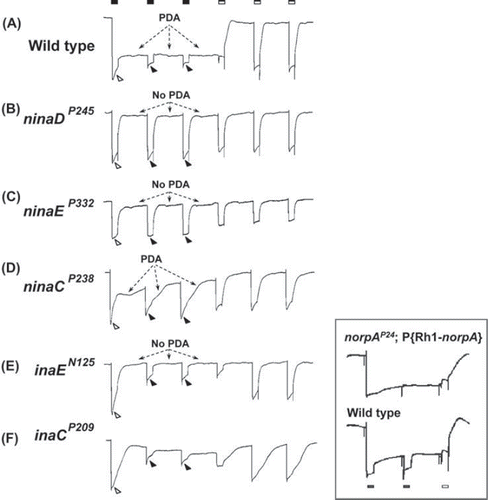
Figure 2. Intracellularly recorded photoreceptor responses of ina mutants and wild type. Photoreceptor responses to 20-s white stimuli recorded intracellularly from flies of the indicated genotypes are presented superposed on each other. In wild type, the response rapidly declines to a steady-state level (filled arrowhead), which depends on the intensity of the light stimulus, and remains there for the rest of the stimulus. This is a wellknown adaptation response of photoreceptor to the light. In the ina mutants, on the other hand, the decline in amplitude (inactivation)that began at the onset of stimulus continues throughout the stimulus with the time course and the extent of decay depending on the mutant. The kinetics of decay can be complex in some mutants (inaC). Thus, the ina photoreceptor responses tend to terminate prematurely. The premature termination phenotype of a strong ina mutant, such as inaFP106x, closely resembles that of null trp (transient receptor potential) mutants.
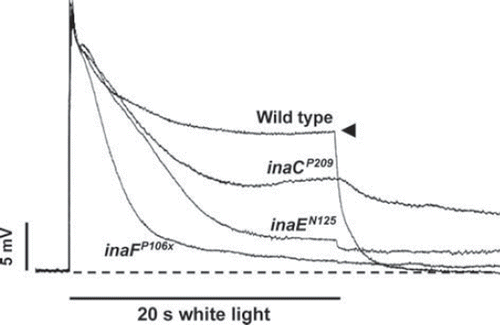
Figure 3. Schematics of putative chromophore biosynthetic pathway. Dietary carotenoids and xanthophils are taken up in the midgut by NINAD and carried by circulation to extra retinal neurons and glia to be taken up there by SANTA MARIA. The dietary β-carotene, once taken up, is reported to be rapidly hydroxylated to form zeaxanthin by unknown mechanisms. In extraretinal neurons and glia, NINAB cleaves and isomerizes zeaxanthin to yield 11-cis (3R)-3-hydroxyretinal and all-trans (3R)-3-hydroxyretinal. Presumably, the chromophore is generated from both products. 11-cis (3R)-3-hydroxyretinal (right branch in ) is converted to its retinol form, 11-cis (3R)-3-hydroxyretinol by a still uncharacterized retinal dehydrogenase. In the mean time, 11-cis (3R)-3-hydroxyretinol may also be generated from all-trans (3R)-3-hydroxyretinal (left branch in ) by conversion to its retinol form and 11-cis isomer. PINTA preferentially binds the retinol form of the retinoids in pigment cells and this binding is required for the chromophore biogenesis, though its precise role is not yet clear. In the final step of chromophore synthesis, 11-cis (3R)-3-hydroxyretinol is converted to its 3S stereoisomer by NINAG to yield 11-cis (3S)-3-hydroxyretinol. This isomerization takes place about the chiral center at the C-3 position of the retinoid ring. C-3 is marked by the red dot and the highlighted OH residue. 11-cis (3R)-3-hydroxyretinol is converted to 11-cis (3R)-3-hydroxyretinal to bind opsin (NINAE) expressed in R1-6 photoreceptors by forming Schiff base linkage with the lysine residue. Inset depicts photo-interconversion of rhodopsin (Rh1) and metarhodopsin (M*).
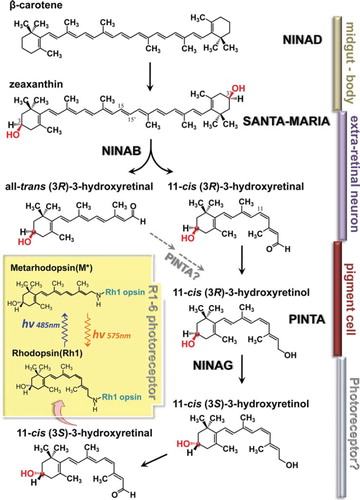
Table 1. nina and ina mutant alleles in the Pak laboratory.
Figure 4. PIP2 hydrolysis in the phototransduction cascade. Upon light stimulation, PIP2 is hydrolyzed by PLCβ (NORPA) to yield diacylglyceorol (DAG) and inositol trisphosphate (IP3). DAG is hydrolyzed by the sn-1 DAG lipase, INAE, to yield 2-monoacylglycerol (2-MAG) and free fatty acid liberated from the sn-1 position of the DAG substrate. 2-MAG is hypothesized to be further hydrolyzed by MAG lipase to yield polyunsaturated fatty acid (PUFA) and glycerol. Note that Drosophila photoreceptor PIP2 is likely to be composed of acyl chains of varying lengths and that the sn-1 and -2 positions are preferentially occupied by the saturated and unsaturated acyl chains, respectively
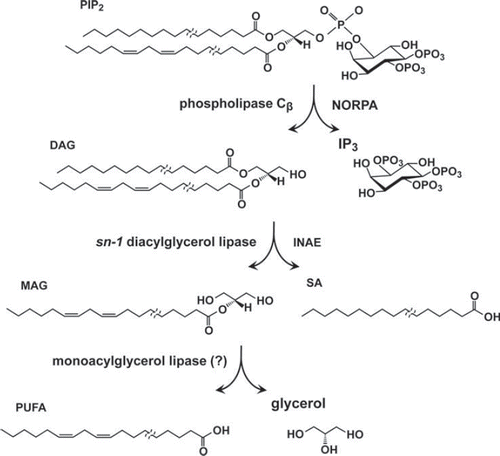