Figures & data
Figure 1 DEAD-box protein structure and RNA unwinding mechanism. (A) Crystal structure of DEAD-box protein Mss116p with bound AMP-PNP (black, at the bottom of the domain interface) and U10 ssRNA (dark blue, at the top of the domain interface).Citation24 The conserved motifs are highlighted and labeled. Domain 1 is on the right.
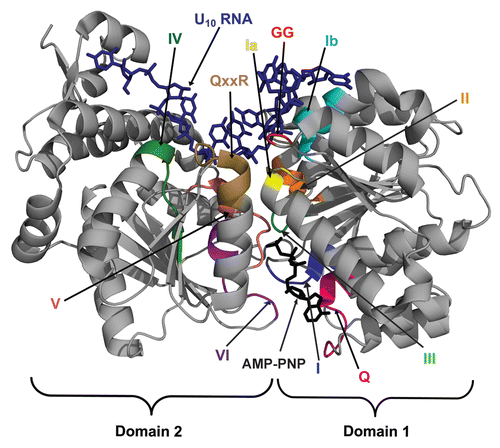
Figure 2 Models for duplex unwinding by conventional helicases and DEAD-box proteins. (A) Conventional helicases load onto single-stranded overhangs of a defined polarity (red) and then translocate into the duplex region (black), displacing the complementary strand. (B) DEAD-box proteins unwind short duplexes in an ATP-dependent but nonprocessive manner by initiating unwinding internally, within a duplex region (black). Some DEAD-box proteins form additional interactions with adjacent single-stranded or double-stranded segments (red), which are suggested to tether the core in position to disrupt nearby helices.
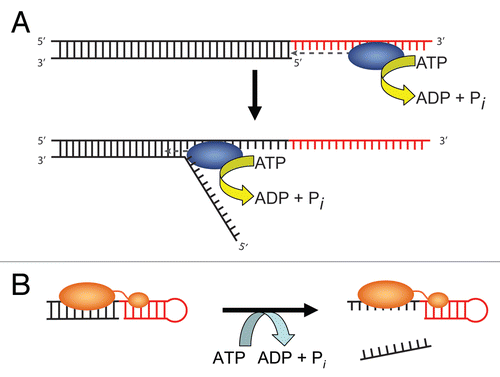
Figure 3 (A) Free energy diagrams comparing RNA and protein folding. Folding of RNA typically proceeds through intermediates of increasing stability, with secondary structure forming first from unfolded structures (U), followed by steps of tertiary contact formation to give a fully folded conformation (F). Many small proteins, on the other hand, do not accumulate intermediates because partially-ordered conformations are less stable than unfolded forms even under conditions that favor global folding. (B) Secondary structures of the native hepatitis delta virus (HDV) and an intermediate with non-native base pairings.Citation68 Nucleotides are color-coded according to their native fold. Alternate base pairings in the HDV intermediate are boxed and labeled.
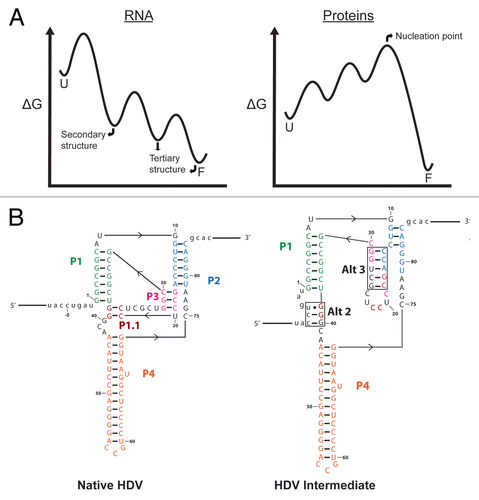
Figure 4 Secondary and tertiary structures of the Tetrahymena group I intron. The shortened “ribozyme” version is shown, in which the exons are removed and the ribozyme forms a core helix, termed P1, with an oligonucleotide that mimics the 5′-splice site. The ribozyme core is highlighted in blue in the secondary structure. Regions of the secondary structure are color-coded according to structural domains, and tertiary contacts are indicated with dashed lines. At the right is a tertiary structure model of the intron,Citation149 with domains in the same colors as in the secondary structure diagram.
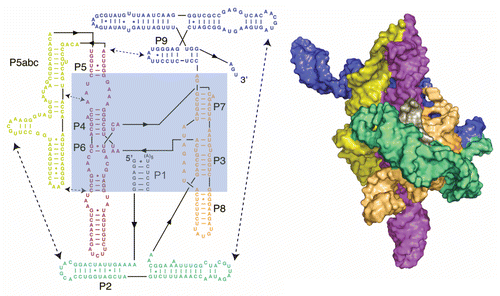
Figure 5 RNA chaperone activity of DEAD-box proteins by non-specific disruption of local structure. The RNA, shown in cartoon form as helical cylinders, represents the Tetrahymena group I intron ribozyme. The ribozyme folds primarily to the long-lived misfolded conformation (bottom; larger rate constants are shown with longer, thicker arrows), which therefore accumulates to high levels despite being less stable than the native state (top). DEAD-box proteins facilitate local structure disruptions of the native and misfolded RNA species in an ATP-dependent manner, without recognizing any structural features of the misfolded RNA. The RNA then folds again without any further influence of the DEAD-box protein. Despite the lack of specific recognition by the DEAD-box protein, the misfolded RNA is disrupted with greater efficiency than the native ribozyme because of its lower stability, and this difference allows the non-specific activity of DEAD-box proteins to accelerate net refolding to a population of predominantly native ribozyme (indicated by arrow at right). Modified from reference Citation125 with permission.
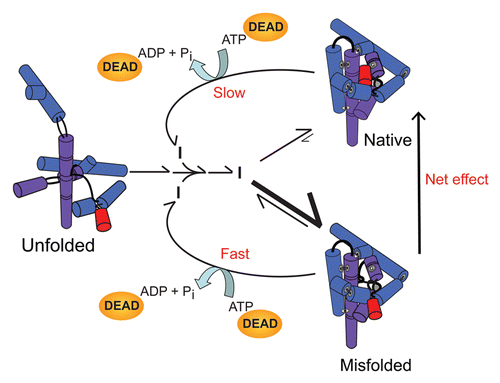
Figure 6 Additional activities of DEAD-box proteins in promoting RNA rearrangements and RNP remodeling. (A) Dbp5p facilitates transport of mRNA from the nucleus to the cytosol. The role of Dbp5p is thought to include removal of the nuclear export factor Mex67p and the nuclear RNA-binding protein Nab2p. Upon protein removal, the mRNA is blocked from re-entering the nucleus. (B) Strand annealing capabilities of DEAD-box proteins. DEAD-box proteins have been shown to accelerate intermolecular duplex formation by two strands of RNA in solution without a requirement for ATP (top). It is possible that this activity is important for intramolecular RNA folding by facilitating formation of local or long-range secondary structure (bottom).
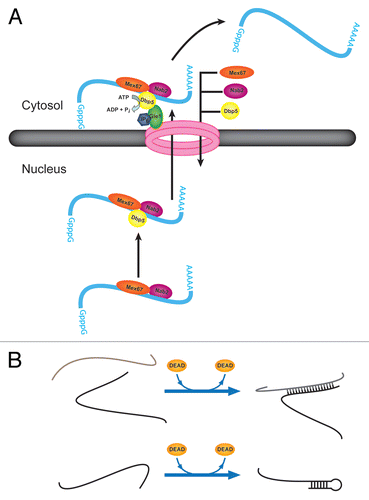