Abstract
We successfully prepared the lithiated H2Ti12O25 sample by the H+/Li+ ion exchange synthetic technique in the molten LiNO3 at 270 °C using H2Ti12O25 as a starting compound. Chemical composition of the obtained lithiated H2Ti12O25 sample was determined to be H1.05Li0.35Ti12O25-δ having δ = 0.3 by ICP-AES and DTA-TG analyses. The H+/Li+ ion exchange was also confirmed by powder XRD, 1H-MAS NMR, and 7Li-MAS NMR measurements. Electrochemical Li insertion and extraction measurements revealed that the initial coulombic efficiency was improved from 88% in H2Ti12O25 to 93% in the lithiated H2Ti12O25 sample. In addition, superior capacity retention properties for the charge and discharge cycling performance and good charge rate capability of the present lithiated H2Ti12O25 were confirmed in the electrochemical measurements. Accordingly, the lithiated H2Ti12O25 is suggested to be one of the promising high-voltage and high-capacity oxide negative electrodes in advanced lithium-ion batteries.
1 Introduction
Lithium titanium oxide Li4Ti5O12 (LTO) with the spinel-type crystal structure has attracted great interest as one of the high voltage oxide negative electrode materials for lithium-ion batteries with long-term cycling, reliable safety, low temperature performance, and good rate-capability [Citation1–Citation4]. LTO exhibits the reversible electrochemical Li insertion and extraction properties at 1.55 V (versus Li/Li+). Because of the structural restriction of the spinel structure, unfortunately, the theoretical capacity of LTO was limited to be 175 mAh g−1, the value of which was about a half of that for graphite for the practically used negative electrode. So, we have investigated to find a novel titanium oxide compound having higher capacity.
Titanium dioxide TiO2 (B) has been investigated as one of the promising candidates [Citation5–Citation7]. This material can be prepared from layered potassium titanate K2Ti4O9 precursor via K+/H+ ion exchange, followed by dehydration, as originally reported by Marchand et al. [Citation5]. Recently, high volumetric reversible capacity up to 475 mAh cm−3 and good cycleability were reported using the micro-size spherical TiO2 (B) sample [Citation8]. On the other hand, another synthetic route to prepare the TiO2 (B) sample using the Na2Ti3O7 precursor was previously reported [Citation9]. Upon heating the parent Na+/H+ ion exchanged H2Ti3O7, the materials demonstrated the multistep weight-loss behavior before the formation of the final product of TiO2 (B) [Citation9,Citation10]. Recently, we successfully prepared single-phase samples of the two intermediate phases, H2Ti6O13 [Citation10,Citation11] and H2Ti12O25 [Citation10], and revealed the better electrochemical properties of H2Ti12O25 than those of TiO2 (B) [Citation10,Citation12].
H2Ti12O25 (HTO) shows similar reversible electrochemical Li insertion and extraction properties at 1.55 V (versus Li/Li+) to LTO and good cycling performance of the relatively higher charge and discharge capacities over 200 mAh g−1 than that for LTO. Although H2Ti12O25 contains hydrogen atoms as a main component element that means the different chemical compositions in H2Ti12O25 and TiO2 (B), the FTIR and 1H-MAS NMR measurements indicated that this compound did not contain water species such as H2O or H3O+ in the structure [Citation10]. However, the irreversible capacity for the initial charge and discharge cycle observed in H2Ti12O25 [Citation10] may be caused by the character of solid acid. For this reason, additional chemical modifications of H2Ti12O25 should be introduced for the purpose of long-term cycling of the battery.
In the present study, we successfully prepared the partial H+/Li+ ion exchanged H2Ti12O25 (L-HTO) sample using the molten LiNO3 for the first time. The chemical composition and the structural information of the H+/Li+ ion exchanged sample were precisely investigated by chemical and spectroscopic analyses. The electrochemical Li insertion and extraction properties of the L-HTO sample were examined in comparison with those for the original H2Ti12O25 (HTO) sample.
2 Experimental procedure
In the present study, the sample preparation was performed by four synthetic steps. The starting Na2Ti3O7 precursor was first prepared by firing Na2CO3 (99.9%, Kojundo Chemical Lab. Co., Ltd.) and TiO2 (99.9%, Kojundo Chemical Lab. Co., Ltd.) in the correct proportion at 800 °C for 20 h in air. The sample was reground and re-heated at 800 °C for 20 h in air, as previously reported [Citation10,Citation13]. Next, the H2Ti3O7 sample was prepared from Na2Ti3O7 via Na+/H+ ion exchange reaction using 0.5 M HCl solution for 5 days at 60 °C. After acidic treatment, the obtained H2Ti3O7 sample was washed with distilled water and ethanol, and then dried at 60 °C for a day in air. Next, the H2Ti12O25 sample was prepared upon heating the H2Ti3O7 sample at 260 °C for 5 h in air. Finally, the H+/Li+ ion exchanged sample was prepared using the molten LiNO3 (99%, Kojundo Chemical Lab. Co., Ltd.) at 270 °C for 10 h in air. After cooling, the obtained lithiated H2Ti12O25 sample was washed with anhydrous ethanol followed by drying at 60 °C for a day in air.
The phase purity of the obtained samples was characterized by powder X-ray diffraction (XRD) measured at room temperature with Cu Kα radiation using a Rigaku RINT-2550V diffractometer (operating conditions: 40 kV, 200 mA) equipped with a curved graphite monochromator. The XRD data were collected for 1 s at each 0.03° step over a 2θ range from 5 to 90°. The particle size and morphology of the samples were observed by scanning electron microscope (SEM, Hitachi S-4800). Chemical composition was analyzed by inductively coupled plasma-optical emission spectroscopy (ICP-OES, Varian 730-ES). Thermogravimetric and differential thermal analyses (TG-DTA) were performed using a Rigaku Thermo Plus TG-DTA apparatus. The H2Ti12O25 and the lithiated H2Ti12O25 samples were heated from room temperature to 1000 °C at a heating rate of 10 °C min−1 in dried air atmosphere. The reference material used was α-Al2O3 powder. The hydrogen contents in the samples were determined by the weight loss below 600 °C due to the dehydration reaction such as the theoretical value of 1.85% for the following dehydration: H2Ti12O25 → H2O↑ + 12TiO2, as previously reported [Citation10].
The solid-state 1H and 7Li magic-angle-spinning nuclear-magnetic-resonance (1H and 7Li MAS NMR) spectra of the H2Ti12O25 and the lithiated H2Ti12O25 samples were measured at room temperature at 300.21 MHz for 1H nuclei and 116.68 MHz for 7Li nuclei with a spinning rate of 10 kHz using a Chemagnetics CMX300 spectrometer.
Electrochemical lithium insertion and extraction experiments were performed using CR2032 coin-type lithium half-cells. The working electrode was made from a mixture of 5 mg of active material, 5 mg of acetylene black, and 1 mg of polytetrafluoroethylene (PTFE) powder. The mixture was pressed on to a Cu mesh having a diameter of 15 mm under a pressure of 20 MPa. The counter electrode was a Li foil having a diameter of 15 mm. The separator was a microporous polypropylene sheet. A solution of 1 M LiPF6 in a 50:50 mixture by volume of ethylene carbonate (EC) and diethylcarbonate (DEC) (LIB grade, Kishida Chemical Co., Ltd.) was used as the electrolyte. The half cells were constructed in an argon-filled glove box. Electrochemical lithium insertion (charge) and extraction (discharge) experiments were performed between 1.0 and 3.0 V at 25 °C after standing 6 h under an open circuit condition. The cells were first charged to 1.0 V at a constant current density of 10 mA g−1, and then discharged to 3.0 V at the same current density, so-called CC charging and discharging mode.
The discharge (Li extraction) characteristics at 25 °C under high current densities up to 3.0 V were evaluated from 10 to 1000 mA g−1 for every three cycles after charging (Li insertion) to 1.0 V at the same current density of 10 mA g−1.
3 Results and discussion
3.1 Synthesis and chemical characterization
summarizes the chemical compositions of the non-lithiated and lithiated H2Ti12O25 samples determined by ICP-OES and TG analyses in the present study. The chemical composition of the non-lithiated H2Ti12O25 sample (HTO) was in good agreement with that for the original report [Citation10]. On the other hand, the weight loss of TG analysis was considerably decreased in the lithiated H2Ti12O25 (L-HTO) sample. It should be noted that the total amount of H and Li in the L-HTO sample was analyzed to be 1.40, that was significantly smaller than 2 in the ideal (H,Li)2Ti12O25 composition. This fact may suggest the oxygen deficiency in this compound such as the chemical formula H1.05Li0.35Ti12O25-δ having δ = 0.3.
Table 1 Chemical compositions of the H2Ti12O25 (HTO) and the lithiated H2Ti12O25 (L-HTO) samples.
compares the powder XRD patterns of the HTO and L-HTO samples. These patterns were qualitatively identified to each other, and can be indexed to the monoclinic Na2Ti12O25-type structure [Citation14], but the peak shifts of some diffraction peaks can be observed in . This fact can be explained by the change of the lattice parameter together with the H+/Li+ ion exchange reaction, such as previously demonstrated in the H+/Li+ ion exchange from H2Ti6O13 [Citation11] to Li2Ti6O13 [Citation15]. On the other hand, the XRD data of the L-HTO sample showed no additional peaks suggesting the impurity phases such as Li2CO3, LiOH, or LiNO3. These facts confirmed that the analyzed Li-content () undoubtedly exchanged the H atoms in the HTO structure.
Fig. 1 Powder XRD patterns for (a) the H2Ti12O25 (HTO) and (b) the lithiated H2Ti12O25 (L-HTO) samples.
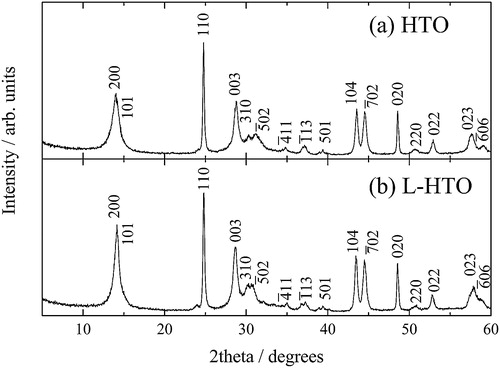
Fig. 2 Magnified powder XRD patterns for (a) the H2Ti12O25 (HTO) and (b) the lithiated H2Ti12O25 (L-HTO) samples.
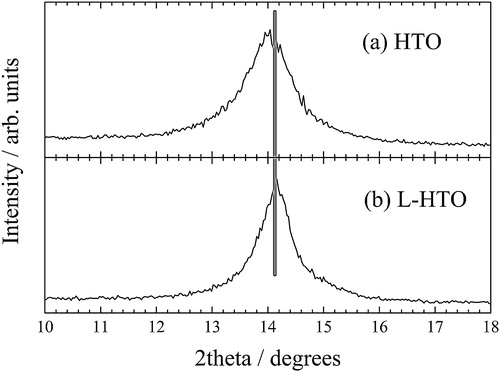
shows typical SEM images of the HTO and L-HTO samples. The particle size of non-treated HTO sample was estimated to be about 1–2 μm, and the particle morphology showed high crystallinity with smooth surface. On the other hand, the particle size of the L-HTO sample remained nearly unchanged from that in the HTO sample, but the particle surface was damaged by the ion-exchange reaction (b).
presents the 1H-MAS NMR spectra of the HTO and L-HTO samples measured at room temperature. As previously reported [Citation3,Citation4], there are two peaks in NMR spectra of both the HTO and L-HTO samples, designated as peak A and peak B at 10 ppm and 6 ppm, respectively. This fact suggests two types of coordination environments around hydrogen in the structure, as previously reported [Citation3,Citation4]. On the other hand, the intensity ratio of two peaks (the ratio of integrated area of peak A and peak B) drastically changed from 6:4 in HTO to 5:5 in L-HTO. This result may be explained by decreasing the hydrogen content situated in the coordination environment corresponding to peak A.
Fig. 4 1H-MAS NMR spectra for (a) the H2Ti12O25 (HTO) and (b) the lithiated H2Ti12O25 (L-HTO) samples.
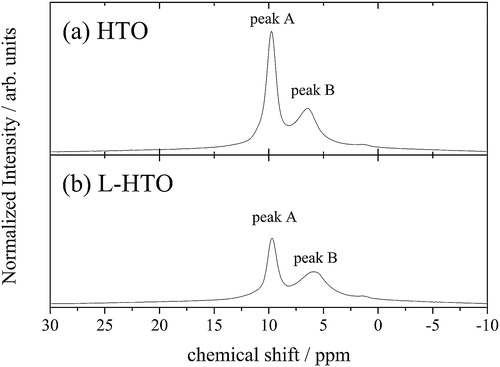
shows the 7Li-MAS NMR spectrum of the L-HTO sample. As can be observed in the magnified spectrum, there is one sharp peak at 0 ppm and additional shoulder peak around −1 ppm with the peak ratio of 9:1. This result may correspond to two types of coordination environments around Li atoms, as in the case of the H atoms. Accordingly, the partial Li+/H+ ion exchange in the present L-HTO sample was confirmed by the chemical and spectroscopic analyses. We tried to prepare the completely Li+/H+ ion exchanged sample by other ion exchange techniques using LiOH or LiI in the high temperature solutions, but have not yet succeeded. These treatments resulted in the formation of Li4Ti5O12 and/or Li2TiO3 as main products.
3.2 Electrochemical properties
shows the initial Li insertion (charge) and extraction (discharge) curves for the HTO and L-HTO samples in the voltage range between 1.0 and 3.0 V (versus Li/Li+) at a constant current density of 10 mA g−1. The initial charge and discharge capacities of the HTO sample were 243 and 215 mAh g−1, respectively, with the initial coulombic efficiency of 88% (a). These results were well consistent with the previous reports [Citation3,Citation4]. On the other hand, the most interesting performance for the present L-HTO sample was that the initial discharge capacity increased to 222 mAh g−1, although the initial charge capacity decreased to 239 mAh g−1 (b). Accordingly, the initial coulombic efficiency was improved from 88% in the HTO sample to 93% in the present L-HTO sample. The irreversible capacity in the HTO sample can be understood that the first Li insertion reaction leads to the formation of SEI on the surface of the HTO electrode together with the decomposition of the electrolyte, and that the subsequent reaction is derived from the Li insertion and extraction in the bulk HTO structure [Citation4]. From this assumption, the decrease of the initial charge capacity in the L-HTO sample may be explained by suppressing the surface reaction of the electrode after H+/Li+ ion exchange in the present study.
Fig. 6 Initial lithium insertion (charge) and extraction (discharge) curves for (a) the H2Ti12O25 (HTO) and (b) the lithiated H2Ti12O25 (L-HTO) samples.
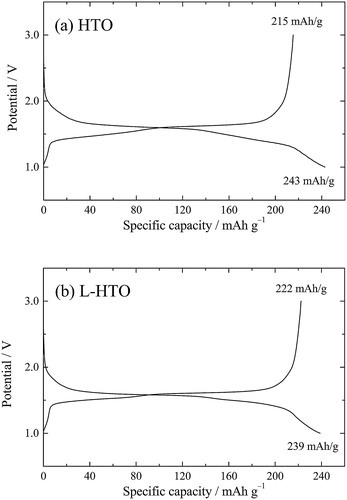
compares the charge and discharge cycling performance of the HTO and L-HTO samples, respectively. These samples delivered superior capacity retention properties. The discharge capacity retention rates were 96% and 97% after 50 cycles for the HTO and L-HTO samples, respectively.
Fig. 7 Charge and discharge cycling performance of (a) the H2Ti12O25 (HTO) and (b) the lithiated H2Ti12O25 (L-HTO) samples for 50 cycles.

shows the differential capacity dQ/dE plots for the first, second, and third cycles in the voltage ranging from 1.0 to 3.0 V with a constant current density of 10 mA g−1 at 25 °C for the HTO and L-HTO samples. It is clearly observed that there are two redox peaks at 1.5 V and 1.6 V for these samples. However, the redox peaks for the first cycle were relatively broad in peak shape and shifted to lower and higher voltages for the HTO sample (a). On the contrary, the L-HTO sample showed relatively sharp redox peak profiles and little voltage change (b). shows the dQ/dE plots for 3–20 cycles for these samples. The L-HTO sample shows superior reversible properties with narrow redox peak profiles in comparison with those for the HTO sample.
Fig. 8 The differential capacity dQ/dE plots for the 1st, 2nd, and 3rd cycles for (a) the H2Ti12O25 (HTO) and (b) the lithiated H2Ti12O25 (L-HTO) samples.

Fig. 9 The differential capacity dQ/dE plots for 3–20 cycles: (a) the H2Ti12O25 (HTO) and (b) the lithiated H2Ti12O25 (L-HTO) samples.
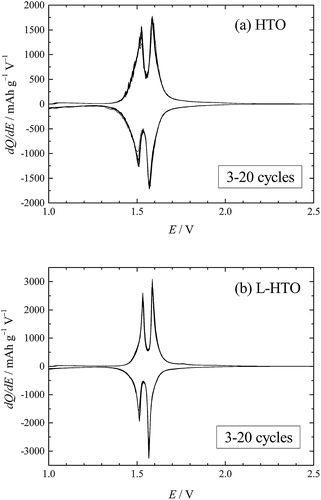
The effect of current density upon discharge (Li extraction) capacity of the HTO and L-HTO samples was examined at 25 °C. compares the rate capabilities for these samples. These samples delivered superior discharge capacity retention properties. Especially, the present L-HTO sample exhibited a remarkable rate capability with little decrease in discharge capacity at rates as high as 1000 mA g−1 (5C rate) having the retaining capacity of 192 mA g−1. From these results, the electrode properties of HTO were successfully improved by the present H+/Li+ ion exchange treatment.
4 Conclusion
In summary, we successfully prepared the partial H+/Li+ ion exchanged H2Ti12O25 (L-HTO) sample using the molten LiNO3 for the first time. The chemical composition and the structural information of the H+/Li+ ion exchanged sample were investigated by the chemical, thermogravimetric, 1H-MAS NMR, 7Li-MAS NMR and powder XRD analyses. The electrochemical Li insertion and extraction properties of the L-HTO sample were examined in comparison with those for the original H2Ti12O25 (HTO) sample. The irreversible capacity for the first charge and discharge cycle was improved by the H+/Li+ ion exchange treatment. We are now attempting to reveal the chemical and structural properties of the L-HTO sample.
Acknowledgment
We express our gratitude to Dr. Y. Sakiyama of Toray Research Center for her experimental help and discussions regarding the 1H-MAS NMR and 7Li-MAS NMR measurements.
Notes
Peer review under responsibility of The Ceramic Society of Japan and the Korean Ceramic Society.
References
- K.M.ColbowJ.R.DahnR.R.HaeringJ. Power Sources261989397402
- N.KoshibaK.TakataM.NakanishiK.ChikayamaZ.TakeharaDenki Kagaku621994970974
- K.KataokaY.TakahashiN.KijimaH.HayakawaJ.AkimotoK.-I.OhshimaSolid State Ionics1802009631635
- N.TakamiK.HoshinaH.InagakiJ. Electrochem. Soc.1582011A725A730
- R.MarchandL.BrohanM.TournouxMater. Res. Bull.15198011291133
- G.ArmstrongA.R.ArmstrongJ.CanalesP.G.BruceElectrochem. Solid State Lett.92006 A139–A143
- M.InabaY.ObaF.NiinaY.MurotaY.OginoA.TasakaK.HirotaJ. Power Sources1892009580584
- N.TakamiY.HaradaT.IwasakiK.HoshinaY.YoshidaJ. Power Sources2732015923930
- T.P.FeistP.K.DavisJ. Solid State Chem.1011992275295
- J.AkimotoK.ChibaN.KijimaH.HayakawaS.HayashiY.GotohY.IdemotoJ. Electrochem. Soc.1582011 A546–A549
- K.KataokaN.KijimaJ.AkimotoSolid State Ionics2522013109115
- J.AkimotoK.KataokaN.KijimaS.HayashiY.GotohT.SotokawaY.KumashiroJ. Power Sources2442013679683
- K.ChibaN.KijimaY.TakahashiY.IdemotoJ.AkimotoSolid State Ionics178200817251730
- K.-J.RangeH.FischerF.KetterlS. Afr. J. Chem.401987233236
- K.KataokaJ.AwakaN.KijimaH.HayakawaK.-I.OhshimaJ.AkimotoChem. Mater.23201123442352