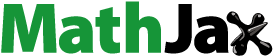
Abstract
Adult male rats were exposed to a 6 Gy single dose from a Cs-137 source. The radio-mitigation effect of poly-MVA was evaluated by daily administration of 2 ml/kg of body weight immediately after irradiation for two weeks. The morphological changes in the red blood cells were studied. The osmotic fragility and rheological properties of blood, the alteration in the contents of antioxidant enzymes (glutathione, catalase and superoxide dismutase) and lipid peroxidation in hepatic cells were determined. The results showed that exposure to radiation resulted in significant changes in cellular antioxidant enzymes (GSH, catalase and SOD) and a decrease in the blood Bingham viscosity, yield stress and aggregation index. Furthermore, it induced a slight increase in the average osmotic fragility of red blood cells accompanied by a decrease in osmotic dispersion, as well as a modification of red blood cell morphology. It also caused a significant increase (75%) in the lipid peroxidation 1 day after exposure to radiation, which persisted until the 14th day recorded after irradiation. Oral administration of poly-MVA after irradiation reduced the radiation-induced damage, as seen in the non-significant change in lipid peroxidation compared to the control. It also resulted in improvement in the observed parameters.
1 Introduction
Accidental exposure to radiation can arise due to factors beyond the control of the operating agencies, e.g., human error, system failure, sabotage, earthquake, cyclone, flood, etc. Incidence of radiological accidents, whether intentional, e.g., associated with military conflict and terrorism, or unintentional, e.g., power plant disasters such as Chernobyl and Fukushima, could lead to large-scale radiation exposure to occupational workers, patients and the public. Timely and effective medical response is a crucial component in reducing radiation harmful effects and mortality. The management of accidental radiation exposure is relatively complicated due to uncertainties in dose, duration, and organs involved in radiation exposure. Treatment of the victim in this case requires information and measurements that may take time to determine the suitable therapeutic strategy for each case. However, after the event of the radiation accident, it will be important to provide an agent that would mitigate the effects of ionizing radiation and can promote or increase the efficiency of the treatment. Ideally, this agent would be long lasting and easily administered (preferably orally) and would possess low toxicity.
Agents that possess radioprotective effects can be classified into three groups according to their action: radioprotectors, adaptogens and absorbents. Radioprotectors are constituted mainly of sulfhydryl compounds and other antioxidants [Citation1]. They act as free radical scavengers that interact with aqueous free radicals or radicals of bio-molecules by donating hydrogen atoms to repair the radical species, thus preventing damage. Adaptogens are substances that can act as stimulators of radio-resistance and offer chemical protection under low levels of ionizing radiation. They include novel immuno-modulators (an agent that augments or diminishes immune responses) and cytokines (number of substances that are secreted by specific cells of the immune system, which carry signals locally between cells, and regulate various inflammatory responses). Some immuno-modulators are well-tolerated and reduce susceptibility to infectious agents, as well as reduce rates of neoplastic transformation. In recent years, radioprotective agents with a novel mode of action have been investigated, in particular, compounds that can affect haematopoietic stem cell regeneration to stimulate the function and regeneration of a stem cell population that has decreased due to radiation induced damage [Citation2]. Absorbents are substances that can protect organisms from internal radiation and chemicals, such as drugs that prevent the incorporation of radioiodine by the thyroid gland and the absorption of radionuclides 137Cs, 90Sr, 239Pu, etc. [Citation3].
According to the administration time, the radioprotective agents have been classified into three categories: prophylactic, mitigators, and therapeutic agents [Citation4]. Prophylactic agents are administered before radiation exposure to prevent radiation damage. A mitigator designates an agent that is administered during or immediately after radiation exposure with the aim of preventing or reducing the action of radiation on tissues before the appearance of symptoms. It is expected to regulate the downstream patho-physiological events of radiation injury. It should act on the radiation injury cascade and thereby prevent the development of further injury. Therapeutic agents are given after the development of clinical symptoms of radiation exposure [Citation5]. Most radio-prophylactic agents are free radical scavengers, anti-oxidants, cytokines, thiols, and steroids [Citation6,Citation7]. They can be classified as radioprotectors and/or adaptogens. Therapeutic agents include suppressors of the renin-angiotensin system and chronic oxidative stress as well as agents such as pentoxifylline to treat radiation fibrosis and growth factors to facilitate recovery from haematological injury [Citation8]. Most radio-protecting agents, whether applied clinically or under research study, are either for prophylaxis or treatment purposes. There are hardly few radio-mitigating agents. However, all radioprotective agents (antioxidants, adaptogens or absorbents) can represent suitable candidates to test their mitigating effects.
Palladium-α-lipoic acid is a complex formulated to act as a non-toxic chemotherapeutic agent for oral administration. It exists in a prescription version called DNA Reductase and is available commercially as a dietary supplement called poly-MVA [Citation9]. The active ingredient in this complex is the palladium-α-lipoic acid polymer, which exists as a trimer of palladium-lipoic acid joined to thiamine in an arrangement that allows it to be both water and lipid soluble. The initials “MVA” stand for minerals, vitamins, and amino acids [Citation10]. Palladium, as a transition metal, serves as a highly efficient aerobic catalyst [Citation11]. Toxicological studies indicated that the LD50 of poly-MVA exceeded 5000 mg/kg, and no mutagenic effect of the combination was observed in the Ames test [Citation12]. In a few recent studies, it showed significant radio-protective and mitigation effects [Citation13–Citation16]. It significantly reduced the γ-radiation-induced mortality in mice and aided recovery from the radiation-induced loss of body weight after 8 Gy exposures. Additionally, the radiation-induced DNA damage in these cells was reduced when poly-MVA was administered to animals exposed to a lethal dose of 8 Gy whole-body γ-radiation [Citation14]. Its administration, for seven days prior to whole body gamma radiation, significantly reduced the damage to cellular DNA in bone marrow and blood leukocytes and prevented the radiation-induced decrease of tissue antioxidant levels [Citation13]. Administration of poly-MVA for 14 days before exposure to 6 Gy gamma radiation, from a Cs-137 source, resulted in normal blood viscosity and yield stress compared to a control group 14 days after exposure, reducing the damage induced by radiation exposure. It also resulted in the normal mean osmotic fragility of red blood cells and reduced lipid peroxidation [Citation15].
The mitigation effect of poly-MVA was tested in a previous work, and the results showed that the administration of poly-MVA for two weeks after whole-body exposure to 6 Gy gamma radiation alleviates the changes in dielectric properties of red blood cells [Citation16]. In the present work, the study of the mitigation effect of poly-MVA on the rheological properties of blood after whole body exposure to gamma radiation was carried out.
2 Materials and methods
2.1 Chemicals
Poly-MVA is a liquid dietary supplement that contains 23.5 mg/ml of lipoic acid-palladium complex, as well as minerals (molybdenum, rhodium and ruthenium), vitamins (B1, B2 and B12), and amino acids (N-acetyl cysteine and formyl methionine), as shown in .
Table 1 Composition of poly-MVA.
Poly-MVA, used in this study, was obtained as a gift from Garnett McKeen Laboratory, Inc., USA. The active ingredient of poly-MVA is palladium α-lipoic acid complex. It presents a three-dimensional structure, with the palladium in the centre of the complex, covalently coordinated with both the oxygen of the lipoic acid carbonyl and the two sulphurs of the thiolane ring and the carboxyl of the pentanoic chain in a 1:1 ratio [Citation10,Citation17,Citation18].
2.2 Animals
Adult male Swiss Albino rats weighing 200 g were used. They were divided into four groups of 18 animals each: the control group, treated group with poly-MVA, irradiated group and treated-irradiated group. Rats were kept under standard conditions along the experimental period. Food and water were supplied daily ad libitum. All animals were housed according to the ethical rules in compliance with institutional guidelines. The conditions were the same for all animals throughout the study. The animals were housed in standard cages (26 cm × 42 cm × 15 cm) with sawdust, at constant room temperature (25 ± 1 °C) and relative humidity (45–55%) with a 12 h light/dark cycle. They were dissected at three time intervals: 1, 7 and 14 days after exposure to radiation.
2.3 Irradiation
Animals were placed in a specially designed, well-ventilated, acrylic pie cage, which holds up to 20 rats. The whole body of the animals was exposed to 6 Gy gamma radiation from a biological irradiator gamma cell-40, cesium-137 source with a dose rate: 0.769 cGy/s (manufactured at the atomic energy agency, Canada) at the National Center for Radiation Research and Technology, Cairo. The dose rate was calibrated at the beginning of the experimental work by the Egyptian High-Dose Reference Laboratory.
2.4 Treatment
Poly-MVA was taken orally with a daily dose of 2 ml/kg of body weight. The rats were divided into three main groups:
1. | Normal control group: the 18 rats were divided into three subgroups of six rats each and kept un-irradiated and un-treated as a normal control group. They were dissected at the same time intervals as the irradiated groups. |
2. | Irradiated group: it included three subgroups according to the dissection time: 1, 7 and 14 days after irradiation. Each irradiated subgroup of rats was composed of six rats. |
3. | Positive control group: poly-MVA was taken daily for two consecutive weeks. The rats were dissected after three intervals: 1, 7 and 14 days during administration. |
4. | The radio-mitigating effect of poly-MVA was examined by daily administration of poly-MVA after irradiation for two consecutive weeks. The animals were dissected at 1, 7 and 14 days after irradiation, with the concomitant administration of poly-MVA. |
2.5 Preparation of samples
Animals were anesthetized by exposure to diethyl ether in a closed container using the open-drop method. The blood samples were withdrawn from the left ventricle of the heart using heparinized needles. After dissection, the liver was washed with isotonic buffered NaCl. 10% of liver samples were homogenized in saline using a Tri-R STIR-R model K41 homogenizer, and then the homogenate was centrifuged at 3000 rpm for 10 min at 4 °C. The supernatant was separated for use in analysis.
2.6 Biochemical assays
Malondialdehyde concentration (MDA), catalase activity (CAT), superoxide dismutase activity (SOD) and glutathione concentration (GSH) were measured in the liver homogenate. MDA was measured by using a thiobarbituric acid assay according to the method of Ohkawa et al. [Citation19]. The obtained results were shown as nmol of malondialdehyde/g of tissue. GSH concentration was measured according to the method of Beutler [Citation20]. It was calculated as μg/g of tissue. CAT activity was measured by catalytic reduction of hydrogen peroxide using the method of Sinha [Citation21]. It was expressed in terms of units of catalase activity that consume 1 μmol of H2O2/min/g of tissue. SOD activity was measured by using the method of Minami and Yoshikawa [Citation22], in unit/g of tissue. One unit of SOD activity is defined as 50% of the inhibitory level of the enzyme in 1 min per gram tissue under the assay condition.
2.7 Haematological analysis
The haemoglobin concentration (Hb) was evaluated using Drabkin's reagent and the haemoglobin standard, which were obtained from EAGLE Diagnostics, USA. The principle of the method involves the oxidation of oxyhaemoglobin, by ferricyanide, to methaemoglobin, and then cyanide converts methaemoglobin to cyanomethaemoglobin [Citation23]. The absorbance of the solutions was recorded at 540 nm, and the concentration of haemoglobin was obtained from the comparison of the value of the absorbance to the calibration curve of the standard haemoglobin.
The determination of the haematocrit (Hct) was performed as follows: blood samples, in a haematocrit microcapillary tube (75 mm/75 μl), were centrifuged for 5 min at 11,500 rpm. Then, the haematocrit values were determined by means of a microcapillary tube reader.
The mean corpuscular volume (MCV), in femto-litres, expresses the average size of the red blood cells (RBCs). It is related to the haematocrit (Hct) by the following relation:
The mean corpuscular haemoglobin concentration (MCHC), in mg/ml, is a measure of the concentration of haemoglobin in a given volume of packed RBCs. It can be calculated as follows:
2.8 Normal red blood cell haemolysis
Normal red blood cells (RBCs) haemolysis was determined by measurement of the haemoglobin released from the cells relative to the total cellular haemoglobin content. Ten microlitres of whole fresh blood was incubated in 5 ml of normal saline for 30 min. The samples were centrifuged at 3000 rpm for 10 min, and the absorbance of the supernatant was measured spectrophotometrically at 540 nm. The percentage of haemolysis was taken against complete blood haemolysis in distilled water [Citation24].
2.9 Rheological properties of blood
The rheological properties were measured by means of a Brookfield DV-III Programmable Rheometer. It is a cone-plate viscometer that measures the fluid parameters of shear stress and viscosity at given shear rates. The body temperature varies from 29 °C at the surface to 37 °C in the blood stream. RBCs’ properties are well known to be temperature-dependent; thus, statistically significant differences were present in a wider range of temperatures between 25–37 °C [Citation25]. In this study, the temperature was set at 35 °C. The applied shear rate ranged from 7.5 to 375 s−1. The data were collected from the rheometer using the software program “Rheocalc for Windows”.
2.10 Osmotic fragility measurements
The process of osmosis was studied by placing the red blood cells (RBCs) in solutions of different tonicity. The osmotic lysis of RBCs can be detected by the release of haemoglobin (Hb) into the extracellular fluid. The amount of Hb appearing in media of different ionic strength was determined spectrophotometrically at 540 nm to characterize the degree of haemolysis according to the method reported by Dacie and Lewis [Citation23].
2.11 Morphological analysis
The morphology of RBCs was studied using a scanning electron microscope (SEM) according to Ross et al. [Citation26] as follows: three drops of RBCs’ suspension were added directly to 5 ml of 2.5% glutaraldehyde in 0.1 M cacodylate buffer (pH 7.4). Fixation was allowed to proceed for at least 24 h before processing. The cells were washed twice in cacodylate buffer, dehydrated with two washes in 70% ethanol, washed twice in 95% ethanol, twice in absolute ethanol and twice in acetone. One drop of the cell suspension was applied to an acetone-washed coverslip and allowed to dry. The cover slip was fixed to an aluminium stub using a colloidal silver adhesive and gold coated using a sputter coater (SPI). Electron microscopy was carried out using a Jeol Model JSM-5400 scanning electron microscope at the National Center for Radiation Research and Technology (NCRRT).
2.12 Statistical analysis
In this study, the values are expressed as the mean values ± standard deviation. The significance of the differences between the values of the treated groups and the control and of the irradiated groups was evaluated by using the Student t-test; values with p < 0.05 were considered as statistically significant [Citation27].
3 Results
3.1 Biochemical assays
The concentration of MDA increased significantly after the 1st day of exposure to radiation by 75.57% (p = 0.001) above the control and continued to increase up to the 14th day, reaching 84.11% (p = 0.008). The response of the three considered antioxidant enzymes in this study differed as a result of exposure to radiation. The concentration of the GSH decreased significantly, from the 1st day after exposure by 8.43% (p = 0.011) of the control value until the 14th day, reaching 11.97% of the control (p = 0.001). SOD decreased significantly (p = 0.257 × 10−2), after 1 day of exposure, by 19.44% of the control value and continued to decrease rapidly until the 14th day, reaching 76.93% (p = 0.891 × 10−8). Meanwhile, the CAT exhibited a different trend, as it showed a significant increase of 36.51% (p = 0.0094) of the control after 1 day of exposure to radiation and reached 17.74% (p = 0.0214) by the 14th day ().
Fig. 1 The malondialdehyde concentration (MDA), glutathione (GSH), catalase (CAT) and superoxide dismutase (SOD) enzymes in control, treated, irradiated and treated-irradiated groups at time intervals of 1, 7 and 14 days.
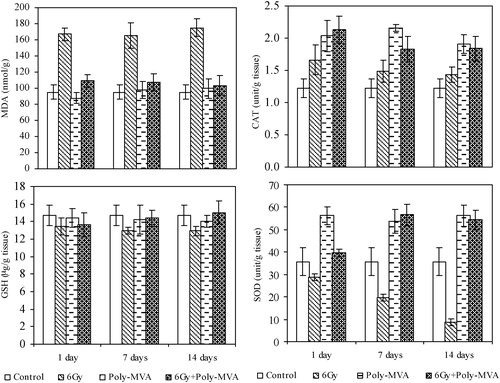
Poly-MVA resulted in non-significant changes in the concentration of MDA from the 1st to the 14th days after administration compared to the control group (p = 0.156, 0.347 and 0.247). CAT and SOD exhibited significant increases from the control in the overall period of administration of approximately 70% (p = 0.848 × 10−3) and 55% (p = 1.22 × 10−4), respectively, while GSH did not show significant changes during the same period (p = 0.284, 0.292 and 0.092 at 1st, 7th and 14th days, respectively) ().
Comparing the effect of poly-MVA after the exposure to 6 Gy of the irradiated groups showed that:
a) | It resulted in a significant decrease in the MDA concentration of 34.5% (p = 0.007) after the 1st day of exposure and reached 40.8% (p = 0.009) on the 14th day after exposure. |
b) | The GSH concentration showed a gradual increase from 0.93% (p = 0.427), after the 1st day of exposure, to 10.85% (p = 0.012) and 16.0% (p = 0.004), at the 7th and 14th day, respectively. |
c) | The CAT increased significantly after 1 day of radiation exposure by 28.08% (p = 0.027), persisting to the 14th day (p = 0.008) with the same ratio. |
d) | The SOD increased significantly by 37.7% (p = 0.0188) after the 1st day and increased highly significantly by 187.9% (p = 0.056 × 10−2) and 561.6% (p = 0.117 × 10−4) at the 7th and the 14th day, respectively (). |
Comparing the effect of poly-MVA after the exposure to 6 Gy of the control group showed that:
a) | The MDA concentration increased after the 1st day of exposure by 14.99% (p = 0.204), while it decreased to 12.74% (p = 0.056) and 8.98% (p = 0.229) by the 7th and 14th day, respectively. |
b) | The GSH concentration showed non-significant changes (p = 0.058, 0.278 and 0.329 on the 1st, 7th and the 14th days, respectively). |
c) | The CAT increased significantly, after 1 day of radiation exposure, by 77.5% (p = 0.0003) and decreased to 49.85% (p = 0.039) and 51.3% (p = 0.417 × 10−3) by the 7th and 14th day. |
d) | The SOD increased non-significantly by 10.94% (p = 0.187) after the 1st day and increased highly significantly by 58.81% (p = 0.131 × 10−2) and 52.65% (p = 0.699 × 10−3) at the 7th and 14th days, respectively (). |
3.2 Haematological analysis
The exposure to 6 Gy resulted in significant decrease, compared to the control group, in the haemoglobin concentration, starting with an 8.9% (p = 0.448 × 10−3) decrease after the 1st day and continuing to 24.9% (p = 0.018) and 47.3% (p = 1.43 × 10−10) at the 7th and 14th days, respectively. The haematocrit showed a significant decrease from the control group of 7.78% (p = 0.011) after the 1st day and continued to decrease to 13.42% (p = 2.93 × 10−4) and 42.14% (p = 3.18 × 10−8) at the 7th and 14th days, respectively. The MCV increased significantly by 15.46% (p = 0.302 × 10−2) after the 1st day compared to the control group, then by 9.79% (p = 0.018) and 7.06% (p = 0.037) at the 7th and 14th days, respectively. The MCHC exhibited a significant decrease from the control group of 4.7% (p = 0.004) after the first day and continued to decrease to 6.4% (p = 0.002) and 7.85% (p = 0.032) after the 7th and 14th days, respectively (). The administration of poly-MVA resulted in non-significant changes in the haematological parameters, except the haematocrit value, which showed a significant decrease of 10.26% (p = 0.003) at the 14th day of treatment ().
Fig. 2 The haemoglobin concentration (Hb), haematocrit (Hct), mean corpuscular volume (MCV) and mean corpuscular haemoglobin concentration (MCHC) for control, treated, irradiated and treated-irradiated groups at time intervals of 1, 7 and 14 days.
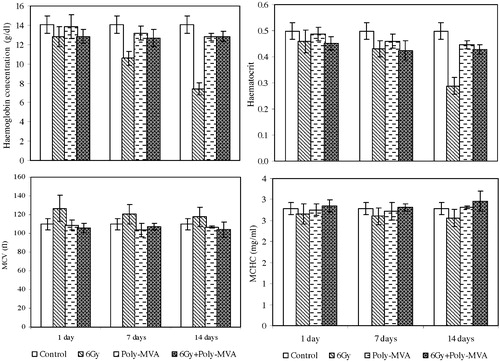
The treatment with poly-MVA after exposure to 6 Gy did not show significant changes in the haemoglobin concentration at the 1st day after exposure compared to the irradiated group (p = 0.466), while it increased significantly by 12.9% (p = 0.004) and 73.2% (p = 0.35 × 10−3) at the 7th and 14th days after exposure. However, this increase in the haemoglobin concentration was significantly lower than the control group, by approximately 9% (p = 0.061, 0.020 and 0.029) all over the considered period. The haematocrit value exhibited an increase from the irradiated group of 1.6% at the 1st and 7th days (p = 0.325 and 0.352), and then it showed a significant increase at the 14th day of 48.44% (p = 0.788 × 10−7). Meanwhile, these values were 9.25% (p = 0.208 × 10−3), 14.78% (p = 0.134 × 10−2) and 14.11% (p = 0.2236 × 10−2) below the control values at the 1st, 7th and 14th days after exposure, respectively. The mean corpuscular volume and mean corpuscular haemoglobin concentration showed non-significant changes from the control group all over the experimental period. Compared to the irradiated group, the mean corpuscular volume showed a significant decrease of 16.65% (p = 0.777 × 10−3), 11.34% (p = 0.933 × 10−3) and 11.5% (p = 0.0123) at the 1st, 7th and 14th days, respectively, after exposure. The mean corpuscular haemoglobin concentration increased by 7.32% (p = 0.0443), 7.87% (p = 0.378 × 10−2) and 14.13% (p = 0.53 × 10−2) at the 1st, 7th and 14th days, respectively, after exposure ().
3.3 Normal haemolysis
The exposure to 6 Gy gamma radiation resulted in an increase in the RBCs’ normal haemolysis of 23.99% (p = 0.546 × 10−3) at the 1st day after exposure that continued to increase to 113.86% (p = 2.26 × 10−4) and 198.9% (p = 0.0084) at the 7th and 14th days after exposure, respectively, as shown in .
Fig. 3 Normal haemolysis of control, treated, irradiated and treated-irradiated groups at time intervals of 1, 7 and 14 days.
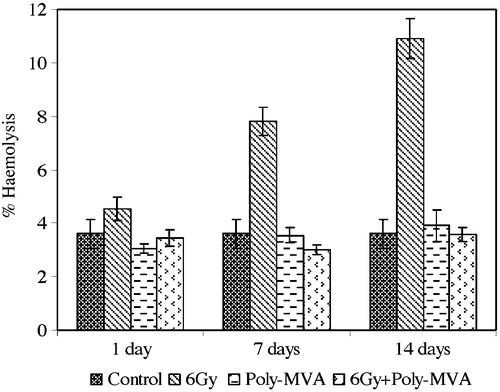
The administration of poly-MVA resulted in non-significant changes in the normal haemolysis compared to the control group (p = 0.156, 0.347 and 0.247 at the 1st, 7th and 14th days after radiation exposure, respectively) ().
The treatment with poly-MVA after exposure to radiation decreased the normal haemolysis by 23.6% (p = 0.0462) at the 1st day, compared to the irradiated group, and continued to decrease, reaching 61.5% (p = 0.2139 × 10−3) and 67.07% (p = 0.8298 × 10−2) at the 7th and 14th days after radiation exposure, respectively (). These values showed non-significant changes from the control groups (p = 0.204, 0.056 and 0.229 at the 1st, 7th and 14th days after radiation exposure, respectively).
3.4 Osmotic fragility
Osmotic fragility can be defined as the sensitivity to change in the osmotic pressure characteristic of red blood cells. It is considered to be a function of the osmotic pressure gradient between intra- and extracellular media, initial surface area to volume ratio, membrane tension of haemolysis and ionic content of the cell [Citation28]. It has been found to be altered in various pathological conditions, such as liver and blood diseases. Thus, the osmotic fragility test was considered as a potential marker for oxidative stress [Citation29].
The quantitative measurements (degree of haemolysis versus decreasing NaCl concentration) are plotted on a graph called the fragility curve, as shown in [Citation30]. The experimental curves were normalized to 100% haemolysis to facilitate the comparison between different samples without the interference of the haematocrit changes. The fragility curve can be evaluated by the following parameters:
1. | Average osmotic fragility (H50): the NaCl concentration producing 50% homolysis. The shift of the fragility curve towards a lower NaCl concentration indicates a decrease in the average osmotic fragility and vice versa. |
2. | Dispersion of haemolysis (S): defined as the difference between the maximum and minimum values of NaCl concentration, at which the rate of haemolysis (dH/dC) = 0. High values of S correspond to isohaemolysis (homogenous RBC population), and low values of S to anisohaemolysis (heterogeneous RBC population) [Citation31]. |
The exposure to 6 Gy gamma radiation resulted in a non-significant increase in the average osmotic fragility from the control group (p = 0.13, 0.17 and 0.06), while the dispersion of haemolysis showed a non-significant change at the 1st (p = 0.13) and 7th (p = 0.29) days after exposure and then increased significantly by 20.76% (p = 0.001) at the 14th day ( and ).
Fig. 5 Average osmotic haemolysis of control, treated, irradiated and treated-irradiated groups at time intervals of 1, 7 and 14 days.
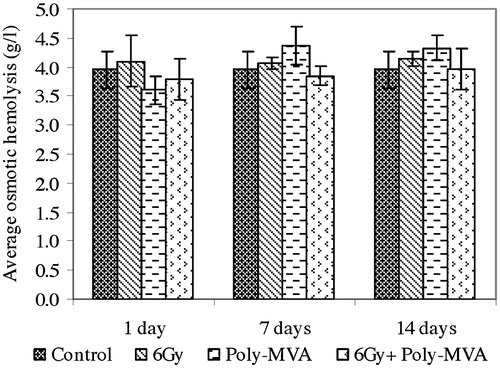
Fig. 6 Dispersion of haemolysis of control, treated, irradiated and treated-irradiated groups at time intervals of 1, 7 and 14 days.
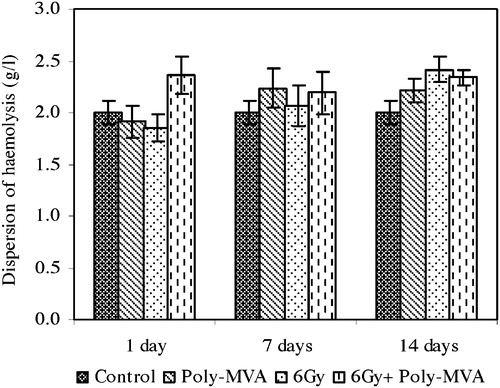
The administration of poly-MVA showed a non-significant decrease from the control in the average osmotic fragility at the 1st day (p = 0.13), which changed to a significant increase at the 7th (p = 0.175 × 10−3) and 14th (p = 0.0132) day (). Meanwhile, the dispersion of haemolysis showed non-significant changes all over the period of administration (p = 0.26, 0.08 and 0.44) ().
The treatment with poly-MVA after irradiation resulted in a non-significant change from the control in the average osmotic fragility (p = 0.17, 0.26 and 0.48), while the dispersion of haemolysis increased significantly by 18% (p = 0.03, 0.0047 and 0.03) all over the period of administration ( and ).
3.5 Rheological analysis
The change in viscosity with shear rate gives the flow curve (), which is characterized by two regions: the low shear rate region (up to 100 s−1) and high shear region (from 100 s−1 up to the shear rate at which no change in viscosity is obtained).
For analysis of the flow curve, the Bingham plastic model () was applied to calculate the yield stress (Fo) and viscosity (η) as follows:where F is the shear stress (dyne/cm2) and D is the shear rate (s−1).
Because the blood viscosity at low shear rate is greatly affected by the RBCs’ aggregation, the ratio of viscosities at 20 and 100 s−1 can be regarded as quantitative characteristics of the RBC aggregation efficiency (Aggregation index) [Citation32].
The exposure to 6 Gy resulted in a significant decrease in the Bingham viscosity of 9.02% (p = 0.059 and 0.031) after 1 and 7 days and reached 24.5% (p = 0.001) at the 14th day (). The yield stress showed a significant decrease from the control of 16.97% (p = 0.032), 32.96% (p = 0.048) and 61.4% (p = 0.334 × 10−4) at the 1st, 7th and 14th days after exposure, respectively, as shown in . The aggregation index decreased by 3.5% (p = 0.148) at the 1st day and continued to decrease, reaching 9.8% (p = 0.037) and 28.6% (p = 0.383 × 10−3) at the 7th and 14th days, respectively ().
Fig. 9 Bingham plastic viscosity of control, treated, irradiated and treated-irradiated groups at time intervals of 1, 7 and 14 days.
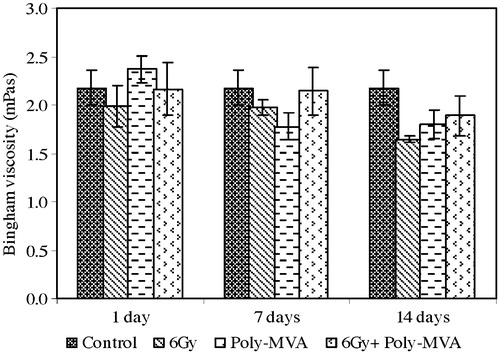
Fig. 10 The yield stress of control, treated, irradiated and treated-irradiated groups at time intervals of 1, 7 and 14 days.
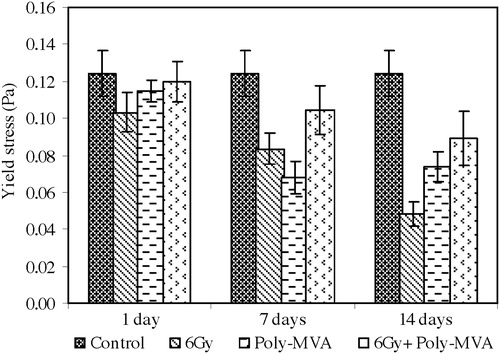
Fig. 11 The aggregation index of control, treated, irradiated and treated-irradiated groups at time intervals of 1, 7 and 14 days.
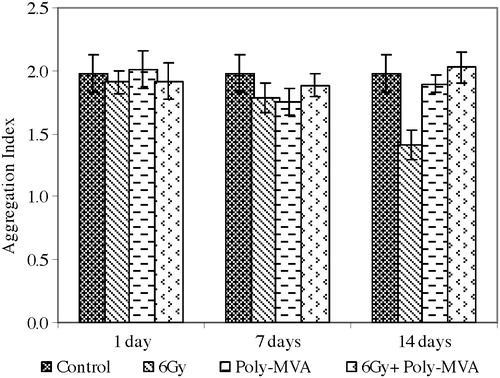
The administration of poly-MVA resulted in a non-significant increase in the Bingham viscosity at the 1st day (p = 0.073), which became a significant decrease of 18% (p = 0.039 and 0.033) at the 7th and 14th days (). The yield stress showed a significant decrease from the control of 3.8% (p = 0.026) at the 1st day and 41% (p = 0.825 × 10−6 and 0.407 × 10−5) at both the 7th and 14th days (). The aggregation index did not show a significant change from the control ().
The treatment with poly-MVA after irradiation resulted in a non-significant change in the Bingham viscosity from the control (p = 0.398, 0.124 and 0.084), while it showed a significant increase of 9% (p = 0.028, 0.038) at the 1st and 7th days and reached 14.7% (p = 0.039) at the 14th day compared to the irradiated group (). The yield stress exhibited a non-significant decrease from the control at the 1st day (p = 0.307), which became a significant decrease of 16.15% (p = 0.727 × 10−4) and 28.39% (p = 0.0112) at the 7th and 14th days, respectively (). Compared to the irradiated group, the yield stress showed a significant increase of 15.97% (p = 0.019), 25.07% (p = 0.034) and 85.46% (p = 0.005) at the 1st, 7th and 14th days, respectively. The aggregation index did not show a significant change from the control (p = 0.375, 0.167 and 0.332), while it showed a significant increase from the irradiated group of 5.65% (p = 0.045) and 43.5% (p = 0.001) at the 7th and 14th days, respectively ().
3.6 Morphological analysis
The morphological changes of RBCs are shown in . It shows the scanning electron micrographs of the control, irradiated, treated with poly-MVA and treated with irradiation groups at time intervals of 1, 7 and 14 days.
Fig. 12 The scanning electro-micrograph of red blood cells of (a) control, (b) irradiated, (c) treated with poly-MVA, and (d) treated with irradiation groups at time intervals of 1, 7 and 14 days.
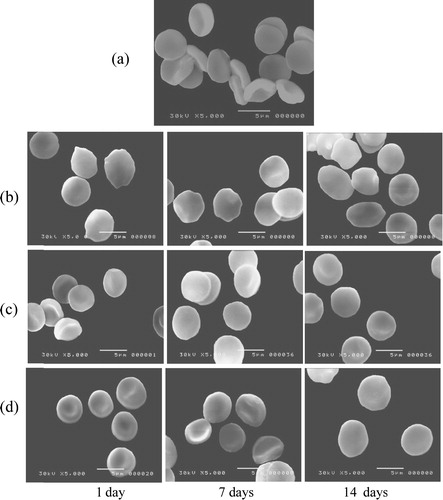
a shows the normal discoid shape of the control RBCs. The exposure to gamma radiation resulted in morphological changes in the RBCs’ membrane, as shown in b, while positive control groups (administered with poly-MVA only) showed a normal biconcave shape (c). Administration of poly-MVA, after exposure to radiation, decreased the formation of abnormal shapes, reducing the radiation induced damage as shown in d.
4 Discussion
4.1 Effect of gamma radiation
Exposure to an acute dose of gamma radiation results in several effects, which can be observed at different levels. It results in oxidative stress due to the production of reactive oxygen species in cells as a result of water radiolysis [Citation33], which leads to lipid peroxidation and oxidation of DNA and proteins, as well as activation of pro-inflammatory factors, which have been observed after both in vitro and in vivo exposures [Citation34]. Lipid peroxidation is commonly measured as a function of malondialdehyde (MDA) concentration as the principal and most studied product of polyunsaturated fatty acid peroxidation [Citation35]. In this study, MDA increased significantly after exposure to an acute dose of gamma radiation, in accordance with a previous study, which showed that doses of 2 and 4 Gy of gamma radiation resulted in an increase in MDA concentration [Citation36].
Biological cells are equipped with several natural enzymatic and non-enzymatic antioxidant defences against these oxidative stresses [Citation37]. Pre-clinical studies have shown the role of anti-oxidant mechanisms in determining the degree of oxidative damage in irradiated tissues [Citation38]. The response of these antioxidant enzymes to ionizing radiation differs according to the exposure dose. Catalase is an inducible enzyme that decomposes H2O2 and is involved in the antioxidant defence mechanisms of mammalian cells. Thus, it is an index of increased H2O2 production [Citation39]. The exposure to gamma radiation of a certain limiting dose may stimulate the activity of the catalase enzyme. This can explain its high activity demonstrated in the livers of irradiated rats compared to controls after whole body exposure to 2 and 4 Gy of gamma radiation [Citation36] and after exposure to 10 Gy [Citation40]. This idea was supported by the direct increase in its biosynthesis 2 h after the exposure of excised chick tissues to 0.5 and 2 Gy [Citation41]. However, increasing the irradiation dose to 15 Gy was shown to decrease its concentration significantly after exposure [Citation42], which could be attributed to the injuries on the hydrogen bonds of its active site. The resulting balance of the two concurrent processes determines the cell's ability to decompose the hydrogen peroxide and restore or deteriorate the radiation-induced damage [Citation41]. In this study, the exposure to 6 Gy resulted also in a significant increase of the catalase after 1 day of exposure to radiation, but it decreased by the 14th day, which can be explained by the start of radiation-induced damage to the enzyme itself.
Glutathione is a water-soluble tripeptide composed of the amino acids glutamine, cysteine, and glycine. It is the most abundant endo-cellular aminothiol, which plays a key role in physiological detoxification [Citation43]. The liver, one of the tissues with the highest content of this tripeptide, is the main tissue participating in glutathione biosynthesis [Citation44]. It is involved in the non-enzymatic removal of reactive oxygen species and also serves as the hydrogen donor in glutathione peroxidase-mediated reactions. The oxidized glutathione is either reduced back to glutathione via glutathione reductase-mediated reactions or exported out of the cells. Therefore, it serves as a measure of the total glutathione capacity of cells at any given time in response to oxidative stress. Superoxide dismutase is another enzyme that is naturally present in human cells that catalyses the conversion of superoxide to oxygen and hydrogen peroxide, acting as an antioxidant during normal conditions and after radiation. It is considered as the primary defensive enzyme against oxygen derived free radical production [Citation45]. Its action needs to be coupled with the glutathione detoxification of hydrogen peroxide to avoid the formation of the highly reactive hydroxyl radical [Citation46]. Thus, it is possible that the enzymes associated with glutathione levels are altered in response to ionizing radiation [Citation47]. In this study, a significant decrease in the concentrations of glutathione and superoxide dismutase was observed from the 1st day after exposure until the 14th day. These results are in accordance with the reported decrease in the hepatic glutathione content following exposure to 2 and 4 Gy gamma radiation [Citation36], as well as the decrease in liver superoxide dismutase activity after exposure to 15 Gy [Citation42].
Decreased tissue glutathione concentrations have been associated with cell damage and depressed immunity [Citation48]. The exposure to low doses, such as from 0.25 Gy to 1 Gy, was shown to induce transformation of red blood cells into echinocytes that are susceptible to destruction and sequestration in small sinuses, thus resulting in low circulating numbers [Citation49]. Additionally, the generation of free radicals during radiation exposure results in destructive processes within both layers of RBCs’ membrane lipids [Citation50] and structural changes in the membrane proteins, as well as a decrease in its intramolecular dynamics [Citation51]. Conformational change of integral membrane proteins could lead to an expansion of one leaflet of the membrane double layer relative to the other, resulting in a shape change [Citation52]. The scanning electron micrographs showed that the exposure to 6 Gy of gamma radiation resulted in modification of the morphology of red blood cells, as shown in the transformation from normal cells to echinocyte and stomatocytes, with a significant increase in the mean corpuscular volume. The transformation to echinocytes and stomatocytes is related to a decrease in the surface area to volume ratio and the membrane permeability, which may lead to a significant increase in the rate of cell haemolysis and change in its behaviour.
The exposure to gamma radiation results in a decrease in the concentration of all cellular components of the blood. This can be due to the direct destruction of mature circulating cells, loss of cells from circulation by haemorrhage, or leakage through capillary walls and reduced cell production [Citation53]. Additionally, the stem cells and early progenitor cells are radiosensitive cells [Citation54]. In this study, acute exposure to 6 Gy of gamma radiation showed a significant decrease in the haemoglobin concentration, and haematocrit decreased after exposure to radiation until reaching 50% of the control value at the 14th day observed. These results may explain the significant decrease in the Bingham viscosity, yield stress and aggregation index of blood, which persisted until the 14th day observed in this study, because the blood viscosity is governed by the physical properties of red blood cells, which constitute 99% of blood cellular components [Citation55]. It is also affected by the changes in the haematocrit, modifications of the membrane skeletal proteins, and the ratio of the RBC membrane surface area to cell volume, cell morphology, and cytoplasmic viscosity, which influence the deformability of RBCs. Additionally, RBC aggregation is mainly determined by plasma protein composition and the surface properties of RBCs [Citation56].
4.2 Effect of poly-MVA
The active ingredient in poly-MVA, i.e., the palladium-lipoic acid polymer, is in the liquid crystal state. It acts as a liquid electrical transistor that transfers electrical current from the cell membrane to the mitochondria, which redistributes the electrical current throughout the cell via the existing electrical pathways. Poly-MVA has a large redox potential, making it a potent antioxidant agent. It oscillates between an oxidized and reduced form as it accepts unpaired electrons and donates them to its enzymatic site; thus, it attracts excess electrons, such as free radicals, and shuttles them to the mitochondria, hence, converting them into energy [Citation9]. It was shown that the oral administration of poly-MVA to male albino rats with 0.38 mg/kg of body weight for a period of 30 days enhanced the activities of Krebs’ cycle dehydrogenases and respiratory complexes in the heart of aged rats. Moreover, it enhanced the activity of catalase, manganese–superoxide dismutase, and glutathione peroxidase, and the level of lipid peroxidation was decreased significantly compared to the aged control. Additionally, because the palladium-α-lipoic acid complex serves as a potent redox molecule, it may facilitate a chain breaking antioxidant effect in the lipid peroxidation process [Citation57]. In this study, the significant decrease in lipid peroxidation in the poly-MVA treated group can be attributed to the enhanced GSH level and CAT activity, which implies that poly-MVA did not exert oxidative stresses after its administration.
The interaction of poly-MVA as a polymer nano-complex with red blood cells’ membranes may occur by unspecific means, including diffusion, trans-membrane channels, and electrostatic, van der Waals, hydration forces, or adhesive interactions [Citation58]. It was shown that there was a significant increase in the relative permittivity and total dipole moment of RBC membranes after poly-MVA administration [Citation59]. Because the increase in the membrane surface charge increases the repulsive force between neighbouring cells and between the cells and vessels’ wall, facilitating their flow, it results in a decrease in blood viscosity and yield stress, with a non-significant change in aggregation index observed in this study. Additionally, the interaction of poly-MVA with cell membranes could result in an increase in membrane elasticity, which explains the increase in membrane osmotic fragility and decrease in viscosity and haematocrit observed at the 14th day of administration.
4.3 Radio-mitigation effect of poly-MVA
The effect of poly-MVA as a radioprotector can be regarded as a result of both its radical scavenging and inducing energy production. Its oxygen radical absorbance capacity (ORAC) value is 5.65 [Citation60], i.e., it is an approximately five times more potent antioxidant than α-lipoic acid. It was demonstrated that this formulation serves as both a highly active free radical scavenger and alternative energy source to brain cells [Citation9]. Due to its antioxidant effect, it acts as a therapy of diseases associated with oxidative stress, either directly as a free radical scavenger or indirectly due to its synergistic action with other antioxidants [Citation18].
The study of its mitigating effect, by its administration after irradiation for two consecutive weeks, was shown to alleviate the radiation-induced damage in the considered parameters. This appeared in the normal results of the mean corpuscular volume and mean corpuscular haemoglobin concentration and no changes in the percentage of haemolysis. Mitigators of radiation injury may target the pathways of radiation injuries to prevent or reduce the expression of toxicity [Citation61]. The radiation injuries can be direct, such as targeting DNA molecules, or indirect by the production of free radicals. As a free radicals scavenger, it can spare an endogenous antioxidant enzyme system and improve the protection against radiation-induced damage, as seen in the normal concentration of GSH and increase in the concentration of SOD reported in this study. This shows the efficiency of the physiological effect of poly-MVA in mitigating the radiation damage induced immediately after exposure, as it acts as antioxidant and adaptive agent. However, some of the studied parameters showed significant changes from control after the post-irradiation treatment with poly-MVA, such as the decrease in yield stress and dispersion of haemolysis, haematocrit and haemoglobin concentration, which may need further investigations.
One more worthy observation in this study is that although the 6 Gy single dose of gamma radiation is considered to be a sub-lethal dose and is known to significantly affect the digestive system, the oral uptake of poly-MVA showed positive results in minimizing the radiation-induced damage, reflecting the efficiency of poly-MVA as a radio-mitigating agent and providing an easy mode of administration.
Acknowledgements
The authors are grateful to Prof. Dr Chirakkal V. Krishnan (Garnett Mckeen Laboratory, NY) for kindly providing the poly-MVA necessary to accomplish this study.
Notes
Peer review under responsibility of Taibah University.
References
- J.C.LiveseyD.J.ReedChemical protection against ionizing radiationAdv. Radiat. Res.131987285353
- Y.M.JoshiT.A.JadhavV.J.KadamRadioprotective-A pharmacological intervention for protection against ionizing radiations: a reviewInternet J. Intern. Med.820105
- D.K.MauryaT.P.A.DevasagayamC.K.KrishnanSome novel approaches for radioprotection and the beneficial effect of natural productsIndian J. Exp. Biol.44200693114
- H.B.StoneJ.E.MoulderC.N.ColemanModels for evaluating agents intended for the prophylaxis, mitigation and treatment of radiation injuriesRadiat. Res.1622004711728
- J.F.WeissM.R.LandauerHistory and development of radiation protective agentsInt. J. Radiat. Biol.852009539573
- J.F.WeissM.R.LandauerRadioprotection by antioxidantsAnn. N. Y. Acad. Sci.89920004460
- S.J.HosseinimehrTrends in the development of radioprotective agentsDrug Discov. Today122007794805
- J.E.MoulderM.MedhoraAdvances in mitigation of injuries from radiological terrorism or nuclear accidentsDef. Sci. J.61201199104
- F.J.AntonawichS.M.FioreL.M.WelickyRegulation of ischemic cell death by the lipoic acid-palladium complex, poly-MVA, in gerbilsExp. Neurol.18920041015
- M. Garnett, Palladium complexes and methods for using same in the treatment of tumors or psoriasis, US Patent 5,463,093 (October 31, 1995).
- S.S.StahlPalladium-catalyzed oxidation of organic chemicals with O2Science309200518241826
- J.BungerJ.StorkK.StalderCyto- and genotoxic effects of coordination complexes of platinum, palladium and rhodium in vitroInt. Arch. Occup. Environ. Health6919963338
- A.MenonC.V.KrishnanC.K.NairProtection from gamma-radiation insult to antioxidant defence and cellular DNA by POLY-MVA, a dietary supplement containing palladium-lipoic acid formulationInt. J. Low Radiat.62009248262
- L.RamachandranC.V.KrishnanC.K.NairRadioprotection by alpha-lipoic acid palladium complex formulation (POLY-MVA) in miceCancer Biother. Radiopharm.252010395399
- S.M.El-MarakbyN.S.SelimO.S.DesoukyH.A.AshryA.M.SallamProphylaxis and mitigation of radiation-induced changes in the electrical properties of erythrocytes by palladium lipoic acid nano-complex (Poly MVA)Rom. J. Biophys.232013171190
- S.M.El-MarakbyN.S.SelimO.S.DesoukyH.A.AshryA.M.SallamEffects of Poly-MVA on the rheological properties of blood after in-vivo exposure to gamma radiationJ. Radiat. Res. Appl. Sci.620132130
- M. Garnett, Palladium complexes and methods for using same in the treatment of psoriasis, US Patent 5,776,973 (July 7, 1998).
- O.CorduneanuA.M.Chiorcea-PaquimaM.GarnettA.M.Oliveira-BrettLipoic acid-palladium complex interaction with DNA, voltammetric and AFM characterizationTalanta77200918431853
- H.OhkawaN.OhishiK.YagiAssay for lipid peroxides in animal tissues by thiobarbituric acid reactionAnal. Biochem.951979351358
- E.BeutlerRed cell metabolismE.ButlerA Manual of Biochemical Methods1963Grune & Stratton, Inc.New York
- A.K.SinhaColorimetric assay of catalaseAnal. Biochem.471972389394
- M.MinamiH.YoshikawaA simplified assay method of superoxide dismutase activity for clinical useClin. Chim. Acta921979337342
- J.DacieS.M.LewisPractical Haematology7th ed.2006Churchill LivingstoneNew York
- I.B.BkaltchevaC.O.OdeyaleB.J.SpargoEffect of alkanols, alkanediols and glycerol on red blood cell shape and hemolysisBiochem. Biophys. Acta128019967380
- O.K.BaskurtF.MatImportance of measurement temperature in detecting the alterations of red blood cell aggregation and deformability studied by ektacytometry: a study on experimental sepsis in ratsClin. Hemorheol. Microcirc.2320004349
- S.R.RossL.WangH.JelinekErythrocyte oxidative damage in chronic fatigue syndromeMed. Res.3820079498
- G.W.SnedecorW.G.CochranStatistical Methods8th ed.1989Iowa State University Press
- S.P.AkesonH.C.MelErythrocyte and ghost cytoplasmic resistivity and voltage-dependent apparent sizeBiophys. J.441983397403
- S.M.Abdel-RaheemZ.M.El-HussieniN.RagabE.Al-GoharyStudy of erythrocyte superoxide dismutase, plasma malondialdehyde and osmotic fragility test in psoriasisJ. Pan-Arab Leag. Dermatol.2020095769
- P.MazeronJ.DidelonS.MullerJ.StoltzA theoretical approach of the measurement of osmotic fragility of erythrocytes by optical transmissionPhotochem. Photobiol.722000172178
- J.F.KergonouC.ThiriotM.BraquetR.DucoussoG.RocquetInfluence of whole body gamma irradiation upon erythrocyte: lipid peroxidation and osmotic fragilityBiochimie681986311318
- N.A.Dobrovol'skiiY.M.LopukhinA.S.ParfenovA.V.PeshkovA blood viscosity analyzerBiomed. Eng.311997140143
- J.P.KamatK.K.BoloorT.P.A.DevasagayamS.R.VenkatachalamAntioxidant properties of Asparagus racemosus against damage induced by γ-radiation in rat liver mitochondriaJ. Ethnopharmacol.712000425435
- W.ZhaoD.I.DizM.E.RobbinsOxidative damage pathways in relation to normal tissue injuryBr. J. Radiol.802007S23S31
- D.del RioA.J.StewartN.PellegriniA review of recent studies on malondialdehyde as toxic molecule and biological marker of oxidative stressNutr. Metab. Cardiovasc. Dis.152005316328
- R.MakhloufI.MakhloufEvaluation of the effect of Spirulina against Gamma irradiation induced oxidative stress and tissue injury in ratsInt. J. Appl. Sci. Eng. Res.12012152164
- B.HalliwellReactive oxygen species and the central nervous systemJ. Neurochem.59199216091623
- E.M.ParkJ.S.ParkT.S.HwangW.C.KimY.M.ParkRole of oxidative stress in the radiation-induced lung pathogenesis in miceJ. Biol. Mol. Biochem.342001544550
- R.MeneghiniIron homeostasis, oxidative stress, and DNA damageFree Radic. Biol. Med.231997783792
- E.M.ParkN.RamnathG.Y.YangJ.Y.AhnY.ParkT.Y.LeeH.S.ShinJ.YuC.IpY.M.ParkHigh SOD and low GPX activities in RBC predict susceptibility of lung cancer patients to radiation pneumonitisFree Radic. Biol. Med.422007280287
- R.FoceaC.NadejdeD.CreangaT.LuchianLow dose X-ray effects on catalase activity in animal tissueJ. Phys.: Conf. Ser.3982012012032
- Y.GuneyN.BukanA.DizmanA.HicsonmezA.BilgihanEffects of two different high doses of radiation on antioxidant system in the liver of guinea pigsExp. Oncol.2620047174
- S.SridharanC.S.ShyamaladeviProtective effect of N-acetylcysteine against gamma ray induced damages in rats – biochemical evaluationsIndian J. Exp. Biol.402002181186
- S.C.LuRegulation of hepatic glutathione synthesis: current concepts and controversiesFASEB J.13199911691183
- M.B.YererH.YapislarS.AydoganO.YalcinO.BaskurtLipid peroxidation and deformability of red blood cells in experimental sepsis in rats: the protective effects of melatoninClin. Hemorheol. Microcirc.3020047782
- I.G.KirkinezosC.T.MoraesReactive oxygen species and mitochondrial diseasesSemin. Cell Dev. Biol.12December (6)2001449457
- S.G.KimS.Y.NamC.W.KimJ.H.KimC.K.ChoS.Y.YooEnhancement of radiation-inducible hepatic glutathione-S-transferases Ya, Yb1, Yb2, Yc1, and Yc2 gene expression by oltipraz: possible role in radioprotectionMol. Pharmacol.511997225233
- C.S.JohnstonC.G.MeyerJ.C.SrilakshrniVitamin C elevates red blood cell glutathione in healthy adultsAm. J. Clin. Nutr.581993103105
- V.D.ZharskayaA.B.ChukhlovinEarly post-radiation changes of red blood cell shape in ratsScanning Microsc.101996279284
- O.I.ShadyroI.L.UrkovaM.A.KiselRadiation-induced peroxidation and fragmentation of lipids in a model membraneInt. J. Radiat. Biol.782002211217
- V.I.Dreval’L.V.SichevskaiaA.O.DoroshenkoA.D.RoshalRadiation induced changes in the structure of erythrocyte membrane proteinsBiofizika452000836838
- J.GimsaC.RiedDo band 3 protein conformational changes mediate shape changes of human erythrocytes?Mol. Membr. Biol.121995247254
- V.NuniaG.SanchetiP.K.GoyalProtection of Swiss albino mice against whole-body gamma irradiation by diltiazemBr. J. Radiol.8020077784
- T.M.FliednerD.H.GraessleV.MeinekeL.E.FeinendegenHemopoietic response to low dose-rates of ionizing radiation shows stem cell tolerance and adaptationDose Response102012644663
- R.PalRheology of concentrated suspensions of deformable elastic particles such as human erythrocytesJ. Biomech.362003981989
- O.K.BaskurtH.J.MeiselmanBlood rheology and hemodynamicsSemin. Thromb. Hemost.292003435450
- N.P.SudheeshT.A.AjithK.K.JanardhananC.V.KrishnanEffect of POLY-MVA, a palladium a-lipoic acid complex formulation against declined mitochondrial antioxidant status in the myocardium of aged ratsFood Chem. Toxicol.48201018581862
- B.M.Rothen-RutishauserS.SchürchB.HaenniN.KappP.GehrInteraction of fine particles and nanoparticles with red blood cells visualized with advanced microscopic techniquesEnviron. Sci. Technol.40200643534359
- N.S.SelimRadioprotective effect of palladium α-lipoic acid complex on the dielectric relaxation and AC conductivity of red blood cellsRom. J. Biophys.222012145161
- F.J.AntonawichP.D.ValanePalladium lipoic acid complex (PdLA): metabolic management for anti-agingProceedings of the Fourteenth International Congress on Anti-Aging Medicine & Regenerative Biomedical TechnologiesAnti-Aging Ther.920071317 (Chapter 3)
- D.CitrinA.P.CotrimF.HyodoB.J.BaumM.C.KrishnaJ.B.MitchellRadioprotectors and mitigators of radiation-induced normal tissue injuryOncologist152010360371