ABSTRACT
1. This study was conducted to investigate the effects of dietary supplementation of manganese (Mn) amino acid complexes on growth performance, Mn deposition, meat quality, breast muscle and bone development of broilers.
2. A total of 504, one-day-old male Arbor Acres broilers were randomly divided into seven treatments; control diet (CON; basal diet, no extra Mn addition), manganese diet (MnN as Numine®-Mn; CON + 40, 80, 120 or 160 mg Mn/kg), manganese-S group (MnS; CON + 120 mg Mn/kg as MnSO4·H2O), manganese-A diet (MnA as Mn from hydrolysed feather meal; CON + 40 mg Mn/kg as MnA).
3. There were no significant differences for average daily gain (ADG) or feed intake (ADFI) among diets during the feed phases (p > 0.05). The FCR in the starter and over the whole period were quadratically affected by dietary MnN dosage and gave the lowest FCR at 80 mg/kg (p < 0.05). The Mn content of thigh muscle, jejunum, heart, pancreas, liver and tibia increased linearly with MnN addition (p < 0.05).
4. For meat quality, MnN significantly increased colour (a*), pH45 min and pH24 h, reduced shear force, drip loss and pressure loss of breast muscle (p < 0.05).
5. Moreover, MnN significantly upregulated MYOD expression at d 21 and SOD expression at d 42, decreased MuRF1 and Atrogin-1 mRNA level at d 42 in breast muscle. Transcriptome analysis revealed that the regulating effect of MnN on muscle development significantly enriched signalling pathways such as adhesion, ECM-receptor, MAPK, mTOR and AMPK. Furthermore, dietary MnN significantly affected tibia length and growth plate development (p < 0.05) and promoted growth plate chondrocytes by increasing SOX-9, Runx-2, Mef2c, TGF-β, Ihh, Bcl-2 and Beclin1 and decreasing Bax and Caspase-3 (p < 0.05) expression which affect longitudinal tibial development.
6. In conclusion, Mn amino acid complexes could improve growth performance, tissue Mn deposition, breast muscle development, meat quality and bone development.
KEYWORDS:
Introduction
The mineral Mn is an essential trace element for animal growth and development (Erikson and Aschner Citation2019). It participates in the synthesis of various enzymes in the body (Hassan, Hassan, and Rehman Citation2020), promotes development of bones (Qi et al. Citation2021; Zofková, Nemcikova, and Matucha Citation2013) and plays an important role in the antioxidant and immune system of animals (Gao et al. Citation2011; Sabaghi, Razmyar, and Heidarpour Citation2021). For fast-growing broilers, demand for Mn is higher than mammals due to the improvement of growth performance based on genetic selection and shorter production cycle in modern livestock (Lu et al. Citation2016). Recent study reported that the Mn requirement for Arbor Acres broilers from 1–3 week of age is 120–130 mg/kg diet, which is twice than the NRC (Citation1994) recommendation (Li et al. Citation2011a). However, there are some unassailable problems with inorganic Mn, such as low absorption rate, interfering with uptake of other minerals (Alagawany et al. Citation2020) and contaminating the environment in livestock manure (Singh, Ghosh, and Haldar Citation2015). Therefore, finding new Mn sources with high efficiency and high utilisation rate has become a goal in the last couple of decades.
Organic forms, like Mn amino acid complexes, have stable chemical properties and higher absorption rates than inorganic Mn (Henry, Ammerman, and Miles Citation1986, Citation1989; F. Wang et al. Citation2012). Numerous studies have demonstrated that organic Mn supplementation improved egg production rate and shell quality (Dos et al. Citation2021; Mwangi et al. Citation2019; Zarghi, Hassanabadi, and Barzegar Citation2023). However, the beneficial effect of organic Mn on mineral metabolism in broilers was rarely elucidated. Moreover, in fast-growing broilers, tibial dyschondroplasia is a common abnormality, whereby about 30% of commercial broilers suffering from varying degrees of lameness (H. Zhang et al. Citation2018). Xia et al. (Citation2022) found that Mn proteinate plays an important role in tibia development and redox homoeostasis in fast-growing broilers. But these effects any underlying mechanisms have not been elucidated.
The aim of this study was to investigate the effects of dietary supplementation of Mn amino acid complexes on growth performance, meat quality, breast muscle and bone development in broilers.
Material and methods
Ethical statement
Experiments were carried out in accordance with the ethical guidelines of Hunan Agricultural University for the care and use of laboratory animals, approved by animal ethics committee of Hunan Agricultural University (HAU ACC 2 022 198).
Animals, diets and experimental design
A total of 504, one-day-old male Arbor Acres broilers (45.0 ± 0.03 g) were randomly divided into seven treatments, namely control (CON) (basal diet, no extra Mn addition), MnN (CON +40, 80, 120 or 160 mg Mn/kg), MnS group (CON +120 mg Mn/kg as MnSO4·H2O), MnA group (CON +40 mg Mn/kg). The MnS and MnA diets acted as positive controls and were Mn amino acid complexes provided by Beijing Deyuanshun Biotechnology Co., Ltd, China. The MnN was Numine® which was chelated with a variety of amino acids (including methionine, lysine, threonine, and glutamic acid etc.) from enzymatically hydrolysed soy protein in a 1:1 molar ratio (moderate chelation strength). The MnA was chelated with a variety of amino acids from hydrolysed feather meal, which had weak chelation strength. Each diet was randomly allocated to six pen replicates containing 12 birds. The feeding trial lasted for 42 d. Birds were fed mash diets to meet the nutrient requirements according to Arbor Acres broiler recommendations (), during the starter (1–21 d) and grower (22–42 d) periods, respectively.
Table 1. Composition and nutrient levels of basal diets (air-dry basis).
The analysed Mn levels in feed were shown in . All chicks were raised following the management guidelines, and the environment was kept at a temperature of 34°C for the first week, gradually dropping to 24°C by the fourth week and afterwards. Feed and water were provided ad libitum during the whole period.
Table 2. Analyzed manganese (mn) contents in experimental diets.
Sample collection and preparation
At 21 and 42 d, one chicken from each replicate was randomly selected for meat quality measurement and tissue sampling and euthanised by cervical dislocation. The breast muscle, thigh muscle, tibia, pancreas, heart, liver and jejunum were removed and stored at −20°C immediately until determination of tissue Mn deposition. The left tibia was taken to measure length and strength and the proximal tibial epiphysis was cut longitudinally to expose the tibial growth plate. Thickness of tibial growth plate was measured using vernier calipers. One-third of tibial growth plates were stored in 4% paraformaldehyde for haematoxylin and eosin (HE) staining, the other tibial growth plates and breast muscles were stored at − 80°C for real-time quantitative polymerase chain reaction (qRT-PCR) analysis.
Growth performance
Birds in each pen replicate were weighed at days 0, 21 and 42 after 12 h fasting to calculate the average daily gain (ADG). The difference between the offered and residual feed for each replicated at each period was recorded to estimate the average daily feed intake (ADFI). Based on ADG and ADFI data, the feed to gain ratio (FCR) was calculated.
Measurement of Mn
The Mn content in the tissues and feed were determined according to the methods of Meng et al. (Citation2021). A sample weighing 0.5–3.0 g (accurate to 0. 001 g) was placed in a conical flask and 10 ml of nitric acid perchloric acid mixture added (9:1). The next day, it was heated on an electric hot plate until the liquid became colourless and transparent or slightly yellow, evolving white smoke. After cooling, ultrapure water was added to make a constant volume and mixed well. Analysis by A3 flame atomic absorption spectrometer was performed (A3F, Persee, Beijing, China).
Meat quality
Samples were taken from the left breast and thigh muscles of broiler. The pH value of the muscles were measured using a pH metre (Mettler Toledo, Zurich, Switzerland) 45 min (pH45) and 24 h (pH24) after slaughter and stored at 4°C. Other meat quality indexes were determined within 45 min of euthanasia. The luminance (L*), redness (a*) and yellowness (b*) of the thigh muscle were measured using a colorimeter (Minolta, Tokyo, Japan). The shear force was measured using a digital tenderness meter (C-LM3B, Tenovo, Beijing, China). The water loss was measured using a pressure gravimetric method according to the methods of Xie et al. (Citation2022).
Bone development indices
The tibia measurements were made using electronic vernier calipers (500-703-20 CD-P20S, Mitutoyo, Japan), with length being the distance between the proximal and distal articular surfaces. The length and width of the tibia were measured in triplicate using calipers accurate to 0. 02 mm. The proximal tibia was cut longitudinally along its apex, and the growth plate width and thickness was measured to calculate its index and cross-sectional area. The growth plate section samples were observed under a microscope (40×; Nikon, Tokyo, Japan), and the thickness of growth plate proliferation zone were measured and analysed with Image J software (National Institutes of Health, Bethesda, MD, U.S.A.), Breaking strength of the tibia was measured using by WD-1 electronic testing (WDS-2000, Weidu, Wenzhou, China) using a three-point bending test method. The machine was equipped with a 50 kg load cell to measure force in compression. The tibia was placed on the horizontal support of the instrument, the span was set to 40 mm, the load speed was set as 10 mm/min, load to fracture of specimen, record fracture strength of tibia (kg). The following calculations were made:
Growth plate width coefficient = growth plate width/tibia length;
Growth plate thickness coefficient = growth plate thickness/tibia length;
Cross-sectional area = growth plate width × growth plate thickness.
RNA Isolation and RT‑qPCR analysis
Total RNA of the breast muscle and tibia was isolated using the AG RNAex Pro reagent (Accurate Bioengineering Co., Ltd., Hunan, China). The concentration and purity of the extracted RNA were measured using a NanoDrop ND-1000 spectrophotometer (Thermo Fisher Scientific, U.S.A.). All primers were obtained from Sangon Biotech Co., Ltd. (Shanghai, China). The primer information for myogenic determining factor (MYOD), myogenin (MYOG), muscle ring finger 1 (MuRF1), muscle atrophy f-box (Atrogin-1), catalase (CAT), superoxide dismutase (SOD), glutathione peroxidase (GPX), SRY-related HMG box-containing 9 (SOX-9), runt-related transcription factor 2 (Runx2), myocyte-specific enhancer factor 2C (Mef2c), transforming growth factor β (TGF-β), Indian hedgehog (IHH), Beclin1 (BECN1), B cell lymphoma/leukemia-2 (Bcl-2), B-cell lymphoma-2 associated X protein (Bax), Caspase-3 and β-actin genes (). Total cDNA was amplified by quantitative RT-PCR reactions in a LightCycler 384 system with SYBR Green I Master. The mRNA levels were calculated using the 2−ΔΔCT method.
Table 3. Primer sequences of genes.
Transcriptome analysis of breast muscle
The total RNA of breast muscles was measured by a quantitative NanoDrop 2000 spectrophotometer using 1.5% agarose gel electrophoresis. After qualified RNA detection, the library was constructed with TruSeq Stranded mRNA LT RNA library preparation kit (Illumina, San Diego, U.S.A.). Library quality was evaluated using the NanoPhotometer, Qubit 2. 0 Fluorometer and Agilent 2100 Bioanalyzer and then sequenced using Illumina HiSeq™ 2500 by the Genedenovo Biotechnology Co., Ltd. The FastQC (v0. 11. 4; Chen et al. Citation2018) was used to check the raw data quality. The rRNA mapped reads were removed by using the Bowtie2 (Version 2. 2. 8; Langmead and Salzberg Citation2012) tool. The software HISAT2–2. 2. 4 (D. Kim, Langmead, and Salzberg Citation2015) was used to align the clean reads to the reference genome. Sample repeatability was tested via principal component analysis. Then, RNA differential expression analysis was performed by DESeq2 (Love, Huber, and Anders Citation2014) software between two different groups. The genes with the parameters of p < 0.05 and |log2FC|>1. 5 were considered differentially expressed genes. Kyoto Encyclopedia of Genes and Genomes (KEGG) is the major public pathway-related database. Pathway enrichment analysis identified significantly enriched metabolic pathways or signal transduction pathways in DEGs compared with the whole genome background.
Statistical analysis
All statistical analyses were performed using SPSS (25. 0, Chicago, IL, U.S.A.) software and values are shown as means with their pooled SEM. Significant differences among different treatment groups were evaluated by one-way analysis of variance (ANOVA) followed by Tukey’s HSD multiple-range tests. Then, the linear and quadratic effects of MnN levels were analysed using regression analysis in SPSS 25. Significance was attained at p < 0.05.
Results
Growth performance
The growth performance of broilers are presented in . At 21, 22–42 and 0–42 d, there was no significant difference in ADFI or ADG among the treatment groups (p > 0.05). FCR in the MnN 80 mg/kg diet from 0–21 d old was significantly lower than that in the MnN 160 mg/kg groups (p < 0.05), and there was no significant difference between the MnN 120 mg/kg and MnS groups (p > 0.05). For the whole period, dietary Mn supplementation reduced FCR quadratically, which was lowest at 80 mg/kg (p < 0.05).
Table 4. Effect of dietary supplementation with MnN on growth performance of broilers.
Mn contents in tissues
The Mn content in tissue is presented in . Compared with CON, the Mn content in breast muscle, thigh muscle, jejunum, heart, pancreas, liver and tibia increased linearly at each stage in broilers with increasing levels of MnN supplementation on d 21 and 42 (p < 0.05). There were no significant differences between MnS and 80 mg/kg MnN groups in Mn content in breast muscle, thigh muscle, heart and tibia on d 21 and thigh muscle, pancreas and tibia on d 42 (p > 0.05). Except for d 21 liver and d 42 heart, there were no significant differences between broilers in the MnA and 40 mg/kg MnN group in other tissue Mn content (p > 0.05).
Table 5. Effect of dietary supplementation with MnN on Mn content in tissue of broilers (mg/kg, fresh matter basis).
Meat quality
The meat data is presented in . Compared with the CON and MnS groups, dietary supplementation of 160 mg/kg MnN significantly increased the a* of breast muscle (p < 0.05) and dietary Mn levels showed a linear increase with breast muscle redness values (p < 0.05). However, MnN supplementation had no effect on the b* and L* values (p > 0.05). Dietary supplementation of MnN significantly increased pH45 min of breast muscle (p < 0.05) compared to the CON group and showed a quadratic effect with pH24 h of breast muscle. Dietary supplementation with 160 mg/kg MnN and 120 mg/kg MnS significantly decreased drip loss of breast muscle relative to CON (p < 0.05), while this was higher in MnA group than MnS group (p < 0.05). Breast muscle pressure loss rate decreased linearly with the addition of MnN (p < 0.05). Thigh muscle a* increases linearly with dietary MnN addition (p < 0.05).
Table 6. Effect of dietary supplementation with MnN on meat quality of broilers at the age of day 42.
Breast muscle development and antioxidation
The breast muscle development of broilers are presented in . On d 21, MnN supplementation linearly increased the mRNA expression level of MYOD (p < 0.05). On d 42, broilers in the MnN groups showed a lower MuRF1 and Attrogin-1 mRNA expression level (p < 0.05) compared with the CON-fed birds.
Table 7. Effect of dietary supplementation with MnN on breast muscle development of broilers.
The breast muscle antioxidants of broilers are presented in . Compared with the CON group, there was no significant difference in CAT, SOD and GPX mRNA expression in the MnN group on day 21 (p > 0.05), but SOD mRNA expression in the MnN 120 and MnS groups and GPX mRNA expression in the MnN 160 group were significantly increased on day 42 (p < 0.05). At day 21, MnN supplementation linearly increased the mRNA expression level of CAT, SOD and GPX (p < 0.05).
Table 8. Effect of dietary supplementation with MnN on breast muscle antioxidant of broilers.
Transcriptome analysis of breast muscle
According to principal component analysis (PCA), three groups of breast muscle samples; CON, MnS and MnN, were classified as shown in and , and showed differences between the diet treatments.
Figure 1. Effect of different dietary manganese sources on breast muscle transcriptome of 21-day-old breast muscle. (a) PCA cluster analysis of transcriptome samples; (b) volcano plot of differential genes; (c) KEGG pathways enriched by differential genes. MnS is MnSO4·H2O; MnN is manganese chelated with a variety of amino acids (including methionine, lysine, threonine, and glutamic acid etc.) from enzymatic hydrolysed soy protein in a 1:1 molar ratio.
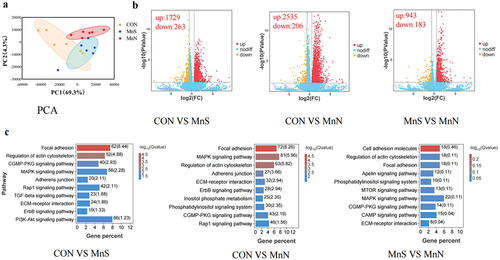
Figure 2. Effect of different dietary manganese sources on breast muscle transcriptome of 42-day-old breast muscle. (a) PCA cluster analysis of transcriptome samples; (b) volcano plot of differential genes; (c) KEGG pathways enriched by differential genes. MnS is MnSO4·H2O; MnN is manganese chelated with a variety of amino acids (including methionine, lysine, threonine, and glutamic acid etc.) from enzymatic hydrolysed soy protein in a 1:1 molar ratio.
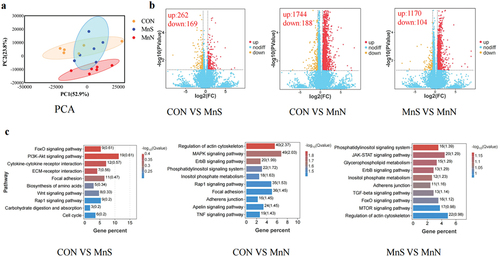
Genes showing significant differences (p < 0.05) and |log2FC|>1. |log2FC|>1.5 were screened as significant differential genes and according to the volcano plot (), it can be seen that, on d 21, there were 1922, 2741 and 1126 differential genes in CON vs. MnS, CON vs. MnN and MnS vs. MnN, respectively, of which 1729, 2535 and 943 up-regulated genes and 263, 206 and 183 down-regulated genes, respectively. On d 42, there were 431, 1932 and 1274 differential genes in CON vs. MnS, CON vs. MnN and MnS vs. MnN, respectively, of which 262, 1744 and 1170 up-regulated genes and 169, 188 and 104 down-regulated genes, respectively.
The KEGG pathway analysis showed that the top 10 differentially enriched pathways were selected by comparing the three groups of breast muscle samples. Differential genes in breast muscle samples at different stages were significantly enriched in signalling pathways such as focal adhesion, regulation of actin cytoskeleton, biosynthesis of amino acids, ErbB, ECM-receptor, MAPK and AMPK.
Tibial variables
The tibial variables of broilers are presented in . Compared to CON, tibial length and strength increased linearly (p < 0.05) and tibial width decreased linearly on d 21 (p < 0.05) with MnN addition. There were no differences in tibial length and width between MnN and MnS treatments (p > 0.05). At d 42, tibial strength showed a linear increase with additional levels of MnN (p < 0.05), but there was no significant difference in tibial length and width among different groups (p > 0.05). Compared to the MnS group, the tibial strength of the MnN 120 and MnN 160 groups was higher on d 42 (p < 0.05).
Table 9. Effect of dietary supplementation with MnN on tibia variables of broilers.
As shown in and , compared with CON MnN supplementation significantly linearly increased the growth plate width, thickness, width index, cross-sectional area and thickness of the proliferative zone on d 21. Addition of 80, 120 or 160 mg/kg MnN significantly improved the width, width index and thickness of the proliferative zone of the growth plate on d 21 (p < 0.05). The growth plate thickness index showed a quadratic increase along MnN dosage in diet on d 21 (p < 0.05). Moreover, diet supplemented with 120 or 160 mg/kg MnN significantly improved the growth plate thickness and growth plate proliferation zone thickness at 42 d old (p < 0.05).
Figure 3. Growth plate morphology on day 21 (a) and 42 (b) (HE staining, 40X). Red lines indicate thickness of growth plate proliferation zone. MnS is MnSO4·H2O; MnN is manganese chelated with a variety of amino acids (including methionine, lysine, threonine, and glutamic acid etc.) from enzymatic hydrolysed soy protein in a 1:1 molar ratio; MnA is chelated with a variety of amino acids from hydrolysed feather meal.
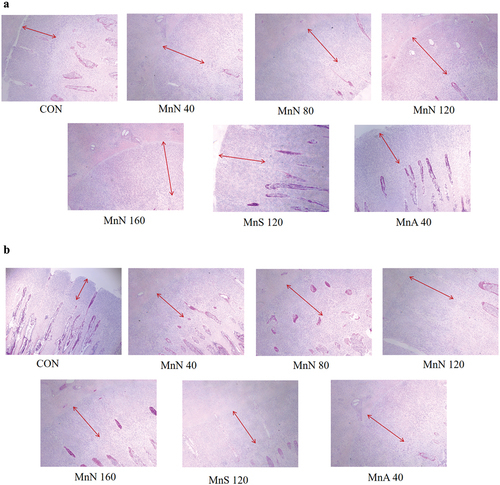
Table 10. Effect of dietary supplementation with MnN on tibia growth plate development of broilers.
As shown in . In samples from d 21, all detected genes in tibia were linearly increased or decreased with dietary MnN dosage (p < 0.05). Broilers in MnS group had a higher Beclin-1 expression in tibia than those fed CON (p < 0.05). The expression levels of SOX-9 in 120 mg/kg MnN group, Mef2c in 120 and 160 mg/kg MnN groups and TGF-β in 80, 120 and 160 mg/kg MnN groups were significantly higher than those fed CON (p < 0.05). Supplementation with MnN, MnS or MnA significantly upregulated Runx-2 expression (p < 0.05), but downregulated Bax and Caspase-3 expression compared to CON group (p < 0.05). At d 21, except for SOX-9 and Bax, other genes showed linearly more or less expression in line with MnN level (p < 0.05). Moreover, addition of 120 mg/kg, 160 mg/kg MnN improved the expression of TGF-β relative with CON and significantly upregulated the Bcl-2 and Beclin-1 expressions (p < 0.05).
Table 11. Effect of dietary supplementation with MnN on growth plate chondrocyte proliferation and differentiation-related molecule expression of broilers.
Discussion
The mineral Mn is an essential element with pleiotropic biological activity involved in various physiological processes, including bone development, antioxidation and growth. However, previous reports about the growth promoting effect of Mn have been inconsistent, where Mn humate supplementation was reported to decrease ADFI of broiler and did not influence body weight at d 14 (Zhao et al. Citation2019). Several researchers have demonstrated that the growth performance of broilers was not affected by adding different doses of Mn sulphate to diets (Li et al. Citation2011b; Brooks et al. Citation2012; Saldanha et al. Citation2020; Sun et al. Citation2021). In addition, Meng et al. (Citation2021) and Bao et al. (Citation2007, Citation2010) found that organic chelate Mn addition in the diet increased ADG and ADFI in broilers and reduced FCR. The present study showed that dietary supplementation with 40–160 mg/kg MnN did not affect ADG and ADFI, but 80 mg/kg in the diet significantly decreased the FCR in the starter phase, and tendency that was seen for the whole growing period. The discrepancies among these studies may have been due to dosage or sources of Mn used. Different forms of organic minerals possess varying chelating strengths, which affect absorption and utilisation rate (Ji et al. Citation2006). Based on these studies, it has been shown that ADG is not a sensitive parameter to predict Mn requirements in broilers when fed corn-soybean meal-based diets.
The source and level of Mn in the diet may directly affect the deposition of Mn in avian tissues. Li et al. (Citation2004) found that Mn content in the heart and bone tissue increased with dietary levels. Similarly, Kim et al. (Citation2022) and Meng et al. (Citation2021) reported that Mn concentrations in serum, liver and tibia increased with higher dietary Mn. This was consistent with the current data, whereby that contents of Mn in broiler tissues increased linearly with supplementation. In addition, in response to deposition in all tissues, tibial Mn was the largest, followed by jejunum, then liver and pancreas and heart (least), which showed that tibial Mn was a better indicator for evaluating Mn bioavailability. In line with the present study, Berta et al. (Citation2004) found that the tibia showed the highest response to Mn, followed by the liver and kidney and then the heart.
Meat quality influences consumer buying behaviour. These results showed that dietary supplementation of MnN increased the redness of breast and thigh muscles, increased breast muscle pH at 45 min and 24 h, decreased shear force and reduced drip and pressure losses. Similarly, Yang et al. (Citation2021) pointed out that Mn supplementation in duck diets significantly increased redness and decreased drip loss and shear force in duck breast muscles. Dietary Mn effects on L*, b* values and WHC of breast or thigh muscles have been demonstrated previously (X. J. Yang et al. Citation2011). These benefits may be related to the conversion process of flesh into meat after slaughter. Anaerobic glycolysis after slaughter produces large amounts of lactic acid and oxidative damage (Lesiów and Xiong Citation2013). As Mn is a component of MnSOD, it is involved in the antioxidant effect of the body (Wołonciej, Milewska, and Roszkowska-Jakimiec Citation2016) and in the tricarboxylic acid cycle (Malthankar et al. Citation2004), energy metabolism and gluconeogenesis (Baly, Keen, and Hurley Citation1986), which can promote myocytes to remove metabolites lactate. In the present study, the addition of MnN in diet improved the quality of breast muscle. In agreement with these results, Flores et al. (Citation2021) found that feeding organic mixtures of zinc and Mn increased breast meat yield. After hatching, growth of skeletal muscle in poultry has been mainly attributed to the enlargement of muscle fibres, a process called hypertrophy (Velleman Citation2007; Zhu et al. Citation2004). This process requires incorporation of more satellite nuclei into the fibres and regulation of protein turnover. The current trial investigated expression of genes related to breast development and protein degradation. Supplementation with MnN significantly increased MYOD mRNA expression on d 21 and decreased MuRF1 and Atrogin-1 mRNA expression on d 42. These results indicated that the improvement due to MnN on breast muscle may result from promoting development and inhibiting protein degradation. This study quantitatively analysed antioxidant genes in breast muscle, which showed that SOD and GPX mRNA expression were significantly increased on d 42. Yang et al. (Citation2021) reported out that dietary Mn significantly decreased MDA content and significantly increased mRNA expression of MnSOD and CAT. In vitro studies have shown that MnCl2 treatment increased MnSOD activity and mRNA expression levels in chicken embryonic cardiomyocytes (R. Wang et al. Citation2022). A few studies have shown that adding Mn sulphate or Met-Mn (Sun et al. Citation2021) to broiler diets can increase MnSOD, CAT and GSH-Px activities, raising T-AOC and decreasing MDA levels in serum, breast and thigh muscles from broilers.
To further explore the underlying mechanism of MnN on muscle growth and meat quality, the transcriptome analysis was conducted on breast muscle of broiler. The KEGG pathway enrichment analysis revealed that signalling pathways, such as adhesion, backbone regulation of actin, biosynthesis of amino acids, ErbB, ECM-receptor, MAPK, mTOR, TGF-β and AMPK, were significantly enriched. Actin is involved in contraction and myoblast differentiation (Sandbo and Dulin Citation2011). The MAPK signalling plays a crucial role in cell proliferation, differentiation, migration, senescence and apoptosis (Sun et al. Citation2015). The ECM receptors are a complex network of structural and functional macromolecules (e.g., laminin, collagen and fibronectin) that play important roles in tissue and organ morphogenesis as well as in maintaining cellular and tissue structure and function (Bosman and Stamenkovic Citation2003). Crittenden and Filipov (Citation2011) showed that Mn-induced activation of MAPK occurs via traditional mechanisms, which perhaps involve the MAPKs farthest upstream (MAP3Ks).
As a key index to evaluate the growth and development of broilers, the tibia isa sensitive factor in broilers. Deficiencies in Mn lead to poor tibial growth and development, leading to become short and thick (Huang et al. Citation2018), narrow growth plate and poor strength (Xia et al. Citation2022). Any deficiency can change tibial morphology, which will inhibit chondrocyte proliferation and induce chondrocyte apoptosis (Liu et al. Citation2015; J. Wang et al. Citation2015). In the current study, dietary supplementation of MnN effectively improved tibial development in broilers at an early growth stage. This may be due to low deposition in the body and rapid growth of bone at an early stage (Chegeni et al. Citation2019). In later growth periods, when feed intake increases, enough Mn was obtained from the diet to meet the needs of bone growth. Bozkurt et al. (Citation2015) confirmed this idea and showed that different levels of Mn added to broiler diets did not affect tibial length at the end of their experiment.
Tibial development is mainly accomplished through endochondral ossification (Farquharson and Jefferies Citation2000), where chondrocytes located in growth plate undergo proliferation, hypertrophy, transdifferentiation, apoptosis and cartilage matrix mineralisation (Chan et al. Citation2021). These processes are co-ordinated by a variety of signalling and transcription factors (Peng et al. Citation2022). The current study found that dietary Mn supplementation significantly affected tibial length as well as the growth plate and RT-qPCR results confirmed that feeding MnN significantly increased SOX-9, Runx-2, Mef2c, TGF-β, IHH, Bcl-2 and Beclin1 and decreased the expression levels of Bax and caspase-3. This demonstrated that the addition of MnN promoted chondrocyte proliferation and endochondral osteogenesis during tibial growth plate hypertrophy. Studies have shown that Mn deficiency leads to disturbances in bone regulatory hormones and metabolic enzymes in serum (Zhaojun et al. Citation2013), affecting tibial development and leading to metaphyseal osteoporosis (Liu et al. Citation2015) and the gene SOX-9 could positively regulate chondrocyte proliferation (Leung et al. Citation2011). This gene deletion blocks cell proliferation, resulting in narrowing of the proliferative zone of the growth plate (Antwi et al. Citation2018). The gene Runx-2 induces Ihh expression in chondrocytes and promotes proliferation of chondrocytes and IHH is able to induce Runx-2 expression in the perichondrium (Laurie et al. Citation2016). In addition, TGF-β plays a positive regulatory role in the early stage of endochondral ossification and has an inhibitory role in the later stage of differentiation to maintain the hypertrophic chondrocyte phenotype (Amarilio et al. Citation2007; Kawakami, Rodriguez-León, and Izpisúa Citation2006). During growth plate development, terminally differentiated chondrocytes must be eliminated to ensure normal thickness is attained. It has been shown in previous studies that apoptosis is involved in the pathogenesis of diseases, such as chondroarthritis (Charlier et al. Citation2016). The study found that the addition of MnN decreased the expression of Bax and caspase-3 and increased the expression levels of Bcl-2 and Beclin1. This indicated that chondrocyte apoptosis was inhibited in the growth plate, which was similar to the findings of Wang et al. (Citation2015). Beclin1 plays an important role in skeletal development and facilitates the mineralisation of bone (Nollet et al. Citation2014). Zhang et al. (Citation2019) reported that the expression of BECN1 decreased in thiram-induced tibial achondroplasia. Therefore, dietary Mn supplementation was able to promote growth plate chondrocyte growth and affected tibial longitudinal development by increasing SOX-9, Runx-2, Mef2c, TGF-β, Ihh, Bcl-2 and Beclin1 and decreasing the expression of Bax and caspase-3.
Conclusions
Dietary supplementation with Mn amino acid complexes can improve growth performance, tissue Mn deposition, breast muscle development, meat quality and bone development.
Authors contributions
The authors LWL and MMM conducted most of the experiments, analysed and interpreted the data and wrote the manuscript. GZ, JX and JLC conducted the research, analysed and interpreted the data. XH and ZHS oversaw the study and reviewed the manuscript. ZHS supervised the study and reviewed the manuscript. All authors read and approved the final manuscript.
Availability of data
All the datasets used and analysed during the current study are included in the manuscript.
Acknowledgments
The authors would like to thank Beijing Deyuanshun Biotechnology Co., Ltd., for providing the necessary Mn amino acid complexes (Numine®-Mn) to conduct this experiment.
Disclosure statement
No potential conflict of interest was reported by the author(s).
Additional information
Funding
References
- Alagawany, M., S. S. Elnesr, M. R. Farag, R. Tiwari, M. I. Yatoo, K. Karthik, I. Michalak, and K. Dhama. 2020. “Nutritional Significance of Amino Acids, Vitamins and Minerals as Nutraceuticals in Poultry Production and Health - a Comprehensive Review.” The Veterinary Quarterly 41 (1): 1–29. https://doi.org/10.1080/01652176.2020.1857887.
- Amarilio, R., S. V. Viukov, A. Sharir, I. Eshkar-Oren, R. S. Johnson, and E. Zelzer. 2007. “HIF1alpha Regulation of Sox9 is Necessary to Maintain Differentiation of Hypoxic Prechondrogenic Cells during Early Skeletogenesis.” Development (Cambridge, England) 134 (21): 3917–3928. https://doi.org/10.1242/dev.008441.
- Antwi, P., C. S. Hong, D. Duran, S. C. Jin, W. Dong, M. DiLuna, and K. T. Kahle. 2018. “A Novel Association of Campomelic Dysplasia and Hydrocephalus with an Unbalanced Chromosomal Translocation Upstream of SOX9.” Cold Spring Harbor Molecular Case Study 4 (3): a002766. https://doi.org/10.1101/mcs.a002766.
- Baly, D. L., C. L. Keen, and L. S. Hurley. 1986. “Effects of Mn Deficiency on Pyruvate Carboxylase and Phosphoenolpyruvate Carboxykinase Activity and Carbohydrate Homeostasis in Adult Rats.” Biological trace element research 11 (1): 201–212. https://doi.org/10.1007/BF02795535.
- Bao, Y. M., M. Choct, P. A. Iji, and K. Bruerton. 2007. “Effect of Organically Complexed Copper, Iron, Mn and Zinc on Broiler Performance, Mineral Excretion and Accumulation in Tissues.” Journal of Applied Poultry Research 16 (3): 448–455. https://doi.org/10.1093/japr/16.3.448.
- Bao, Y. M., M. Choct, P. A. Iji, and K. Bruerton. 2010. “Trace Mineral Interactions in Broiler Chicken Diets.” British Poultry Science 51 (1): 109–117. https://doi.org/10.1080/00071660903571904.
- Berta, E., E. Andrásofszky, A. Bersényi, R. Glávits, A. Gáspárdy, and S. G. Fekete. 2004. “Effect of Inorganic and Organic Mn Supplementation on the Performance and Tissue Mn Content of Broiler Chicks.” Acta Veterinaria Hungarica 52 (2): 199–209. https://doi.org/10.1556/AVet.52.2004.2.8.
- Bosman, F. T., and I. Stamenkovic. 2003. “Functional Structure and Composition of the Extracellular Matrix.” The Journal Pathology 200 (4): 423–428. https://doi.org/10.1002/path.1437.
- Bozkurt, Z., T. Bulbul, M. F. Bozkurt, A. Bulbul, G. Maralcan, and K. Celikeloglu. 2015. “Effects of Organic and Inorganic Mn Supplementation on Bone Characteristics, Immune Response to Vaccine and Oxidative Stress Status in Broiler Reared Under High Stocking Density.” Kafkas Universitesi Veteriner Fakultesi Dergisi 21 (5): 623–630. https://doi.org/10.9775/kvfd.2014.12655.
- Brooks, M. A., J. L. Grimes, K. E. Lloyd, F. Valdez, and J. W. Spears. 2012. “Relative Bioavailability in Chicks of Mn from Mn Propionate.” Journal of Applied Poultry Research 21 (1): 126–130. https://doi.org/10.3382/japr.2011-00331.
- Chan, W., Z. Tan, M. To, and D. Chan. 2021. “Regulation and Role of Transcription Factors in Osteogenesis.” International Journal of Molecular Sciences 22 (11): 5445. https://doi.org/10.3390/ijms22115445.
- Charlier, E., B. Relic, C. Deroyer, O. Malaise, S. Neuville, J. Collée, M. G. Malaise, and D. de Seny. 12 2016. “Insights on Molecular Mechanisms of Chondrocytes Death in Osteoarthritis.” International Journal of Molecular Sciences 17 (12): 2146. https://doi.org/10.3390/ijms17122146.
- Chegeni, M. M., M. Mottaghitalab, S. Moghaddam, and M. Golshekan. 2019. “Broiler Intestine DMT1 Gene Expression and Bone Characteristics, as Affected by in Ovo Injection of Different Forms of Mn.” Italian Journal of Animal Science 18 (1): 1215–1222. https://doi.org/10.1080/1828051X.2019.1646106.
- Chen, S., Y. Zhou, Y. Chen, and J. Gu. 2018. “Fastp: an ultra-fast all-in-one FASTQ preprocessor.” Bioinformatics 34 (17): i884–i890. https://doi.org/10.1093/bioinformatics/bty560.
- Crittenden, P. L., and N. M. Filipov. 2011. “Mn Modulation of MAPK Pathways: Effects on Upstream Mitogen Activated Protein Kinase Kinases and Mitogen Activated Kinase Phosphatase-1 in Microglial Cells.” Journal of Applied Toxicology 31 (1): 1–10. https://doi.org/10.1002/jat.1552.
- dos Santos, E., E. R. Freitas, R. C. Nepomuceno, P. H. Watanabe, D. H. Souza, D. R. Fernandes, F. C. de Abreu, N. G. Do, G. C. Aguiar, and M. de Melo. 2021. “Organic Zinc and Mn and 25-Hydroxycholecalciferol Improves Eggshell Thickness in Late-Phase Laying Hens.” Tropical Animal Health and Production 53 (6): 529. https://doi.org/10.1007/s11250-021-02959-x.
- Erikson, K. M., and M. Aschner. 2019. “Mn: Its Role in Disease and Health.” Metal Ions in Life Sciences 19. https://doi.org/10.1515/9783110527872-016.
- Farquharson, C., and D. Jefferies. 2000. “Chondrocytes and Longitudinal Bone Growth: The Development of Tibial Dyschondroplasia.” Poultry Science 79 (7): 994–1004. https://doi.org/10.1093/ps/79.7.994.
- Flores, K. R., A. Fahrenholz, P. R. Ferket, T. J. Biggs, and J. L. Grimes. 2021. “Effect of Methionine Chelated Zn and Mn and Corn Particle Size on Large White Male Turkey Live Performance and Carcass Yields.” Poultry Science 100 (11): 101444. https://doi.org/10.1016/j.psj.2021.101444.
- Gao, T., F. Wang, S. Li, X. Luo, and K. Zhang. 2011. “Mn Regulates Mn-Containing Superoxide Dismutase (MnSOD) Expression in the Primary Broiler Myocardial Cells.” Biological trace element research 144 (1–3): 695–704. https://doi.org/10.1007/s12011-011-9093-y.
- Hassan, S., F. U. Hassan, and M. S. Rehman. 2020. “Nano-Particles of Trace Minerals in Poultry Nutrition: Potential Applications and Future Prospects.” Biological Trace Element Research 195 (2): 591–612. https://doi.org/10.1007/s12011-019-01862-9.
- Henry, P. R., C. B. Ammerman, and R. D. Miles. 1986. “Bioavailability of Mn Sulfate and Mn Monoxide in Chicks As Measured by Tissue Uptake of Mn from Conventional Dietary Levels.” Poultry Science 65 (5): 983–986. https://doi.org/10.3382/ps.0650983.
- Henry, P. R., C. B. Ammerman, and R. D. Miles. 1989. “Relative Bioavailability of Mn in a Mn-Methionine Complex for Broiler Chicks.” Poultry Science 68 (1): 107–112. https://doi.org/10.3382/ps.0680107.
- Huang, S. C., L. H. Zhang, J. L. Zhang, M. U. Rehman, X. L. Tong, G. Qiu, X. Jiang, et al. 2018. “Role and Regulation of Growth Plate Vascularisation During Coupling with Osteogenesis in Tibial Dyschondroplasia of Chickens.” Scientific Reports 8 (1): 3680. https://doi.org/10.1038/s41598-018-22109-y.
- Ji, F., X. G. Luo, L. Lu, B. Liu, and S. X. Yu. 2006. “Effect of Mn Source on Mn Absorption by the Intestine of Broilers.” Poultry Science 85 (11): 1947–1952. https://doi.org/10.1093/ps/85.11.1947.
- Kawakami, Y., J. rodriguez-león, and B. J. Izpisúa. 2006. “The Role of TGFbetas and Sox9 During Limb Chondrogenesis.” Current Opinion in Cell Biology 18 (6): 723–729. https://doi.org/10.1016/j.ceb.2006.10.007.
- Kim, D., B. Langmead, and S. L. Salzberg. 2015. “HISAT: A Fast Spliced Aligner with Low Memory Requirements.” Nature Methods 12 (4): 357–360. https://doi.org/10.1038/nmeth.3317.
- Kim, M. J., A. Hosseindoust, K. Y. Kim, J. Moturi, J. H. Lee, T. G. Kim, J. Y. Mun, and B. J. Chae. 2022. “Improving the Bioavailability of Mn and Meat Quality of Broilers by Using Hot-Melt Extrusion Nano Method.” British Poultry Science 63 (2): 211–217. https://doi.org/10.1080/00071668.2021.1955332.
- Langmead, B., and S. L. Salzberg. 2012. “Fast Gapped-Read Alignment with Bowtie 2.” Nature Methods 9 (4): 357–359. https://doi.org/10.1038/nmeth.1923.
- Laurie, L. E., H. Kokubo, M. Nakamura, Y. Saga, and N. Funato. 2016. “The Transcription Factor Hand1 Is Involved in Runx2-Ihh-Regulated Endochondral Ossification.” PLOS ONE 11 (2): e150263. https://doi.org/10.1371/journal.pone.0150263.
- Lesiów, T., and Y. L. Xiong. 2013. “A Simple, Reliable and Reproductive Method to Obtain Experimental Pale, Soft and Exudative (PSE) Pork.” Meat Science 93 (3): 489–494. https://doi.org/10.1016/j.meatsci.2012.11.022.
- Leung, V. Y., B. Gao, K. K. Leung, I. G. Melhado, S. L. Wynn, T. Y. Au, N. W. Dung, et al. 2011. “SOX9 Governs Differentiation Stage-Specific Gene Expression in Growth Plate Chondrocytes via Direct Concomitant Transactivation and Repression.” PLOS Genetics 7 (11): e1002356. https://doi.org/10.1371/journal.pgen.1002356.
- Li, S., Y. Lin, L. Lu, L. Xi, Z. Wang, S. Hao, L. Zhang, K. Li, and X. Luo. 2011a. “An Estimation of the Mn Requirement for Broilers from 1 to 21 Days of Age.” Biological Trace Element Research 143 (2): 939–948. https://doi.org/10.1007/s12011-010-8931-7.
- Li, S., L. Lu, S. Hao, Y. Wang, L. Zhang, S. Liu, B. Liu, K. Li, and X. Luo. 2011b. “Dietary Mn Modulates Expression of the Mn-Containing Superoxide Dismutase Gene in Chickens.” The Journal of Nutrition 141 (2): 189–194. https://doi.org/10.3945/jn.110.126680.
- Li, S., X. Luo, B. Liu, T. D. Crenshaw, X. Kuang, G. Shao, and S. Yu. 2004. “Use of Chemical Characteristics to Predict the Relative Bioavailability of Supplemental Organic Mn Sources for Broilers.” Journal of Animal Science 82 (8): 2352–2363. https://doi.org/10.2527/2004.8282352x.
- Liu, R., C. Jin, Z. Wang, Z. Wang, J. Wang, and L. Wang. 2015. “Effects of Mn Deficiency on the Microstructure of Proximal Tibia and OPG/RANKL Gene Expression in Chicks.” Veterinary Research Communications 39 (1): 31–37. https://doi.org/10.1007/s11259-015-9626-5.
- Love, M. I., W. Huber, and S. Anders. 2014. “Moderated Estimation of Fold Change and Dispersion for RNA-Seq Data with DESeq2.” Genome Biology 15 (12): 550. https://doi.org/10.1186/s13059-014-0550-8.
- Lu, L., B. Chang, X. Liao, R. Wang, L. Zhang, and X. Luo. 2016. “Use of Molecular Biomarkers to Estimate Mn Requirements for Broiler Chickens from 22 to 42 D of Age.” The British Journal of Nutrition 116 (9): 1512–1518. https://doi.org/10.1017/S0007114516003640.
- Malthankar, G. V., B. K. White, A. Bhushan, C. K. Daniels, K. J. Rodnick, and J. C. Lai. 2004. “Differential Lowering by Mn Treatment of Activities of Glycolytic and Tricarboxylic Acid (TCA) Cycle Enzymes Investigated in Neuroblastoma and Astrocytoma Cells Is Associated with Mn-Induced Cell Death.” Neurochemical Research 29 (4): 709–717. https://doi.org/10.1023/b:nere.0000018841.98399.ce.
- Meng, T., L. Gao, C. Xie, Y. Xiang, Y. Huang, Y. Zhang, and X. Wu. 2021. “Mn Methionine Hydroxy Analog Chelated Affects Growth Performance, Trace Element Deposition and Expression of Related Transporters of Broilers.” Animal Nutrition 7 (2): 481–487. https://doi.org/10.1016/j.aninu.2020.09.005.
- Mwangi, S., J. Timmons, T. Ao, M. Paul, L. Macalintal, A. Pescatore, A. Cantor, and K. A. Dawson. 2019. “Effect of Mn Preconditioning and Replacing Inorganic Mn with Organic Mn on Performance of Male Broiler Chicks.” Poultry Science 98 (5): 2105–2113. https://doi.org/10.3382/ps/pey564.
- Nollet, M., S. Santucci-Darmanin, V. Breuil, R. al-Sahlanee, C. Cros, M. Topi, D. Momier, et al. 2014. “Autophagy in Osteoblasts Is Involved in Mineralisation and Bone Homeostasis.” Autophagy 10 (11): 1965–1977. https://doi.org/10.4161/auto.36182.
- NRC (National Research Council). 1994. Nutrient Requirements of Poultry. 9th ed. Washington, DC: National Academy Press.
- Peng, G., H. Sun, H. Jiang, Q. Wang, L. Gan, Y. Tian, J. Sun, D. Wen, and J. Deng. 2022. “Exogenous Growth Hormone Functionally Alleviates Glucocorticoid-Induced Longitudinal Bone Growth Retardation in Male Rats by Activating the Ihh/PthrP Signaling Pathway.” Molecular and Cellular Endocrinology 545:111571. https://doi.org/10.1016/j.mce.2022.111571.
- Qi, S. S., M. L. Shao, Z. Sun, S. M. Chen, Y. J. Hu, X. S. Li, J. Chen, H. X. Zheng, and T. L. Yue. 2021. “Chondroitin Sulfate Alleviates Diabetic Osteoporosis and Repairs Bone Microstructure via Anti-Oxidation, Anti-Inflammation and Regulating Bone Metabolism.” Frontiers in Endocrinology (Lausanne) 12:759843. https://doi.org/10.3389/fendo.2021.759843.
- Sabaghi, S., J. Razmyar, and M. Heidarpour. 2021. “Effects of Nano-Mn on Humoral Immune Response and Oxidative Stress in Broilers.” Veterinary Research Forum 12 (4): 487–491. https://doi.org/10.30466/vrf.2020.114233.2716.
- Saldanha, M. M., I. Araújo, M. V. Triguineli, D. P. Vaz, F. Ferreira, J. Albergaria, D. O. Fontes, and L. Lara. 2020. “Relative Bioavailability of Mn in Relation to Proteinate and Sulfate Sources for Broiler Chickens from One to 20 D of Age.” Poultry Science 99 (11): 5647–5652. https://doi.org/10.1016/j.psj.2020.05.006.
- Sandbo, N., and N. Dulin. 2011. “Actin Cytoskeleton in Myofibroblast Differentiation: Ultrastructure Defining Form and Driving Function.” Translational Research 158 (4): 181–196. https://doi.org/10.1016/j.trsl.2011.05.004.
- Singh, A. K., T. K. Ghosh, and S. Haldar. 2015. “Effects of Methionine Chelate- or Yeast Proteinate-Based Supplement of Copper, Iron, Mn and Zinc on Broiler Growth Performance, their Distribution in the Tibia and Excretion into the Environment.” Biological trace element research 164 (2): 253–260. https://doi.org/10.1007/s12011-014-0222-2.
- Sun, Y., S. Geng, T. Yuan, Y. Liu, Y. Zhang, Y. di, J. Li, and L. Zhang. 2021. “Effects of Mn Hydroxychloride on Growth Performance, Antioxidant Capacity, Tibia Parameters and Mn Deposition of Broilers.” Animals (Basel) 11 (12): 3470. https://doi.org/10.3390/ani11123470.
- Sun, Y., W. Z. Liu, T. Liu, X. Feng, N. Yang, and H. F. Zhou. 2015. “Signaling Pathway of MAPK/ERK in Cell Proliferation, Differentiation, Migration, Senescence and Apoptosis.” Journal of Receptor and Signal Transduction Research 35 (6): 600–604. https://doi.org/10.3109/10799893.2015.1030412.
- Velleman, S. G. 2007. “Muscle Development in the Embryo and Hatchling.” Poultry Science 86 (5): 1050–1054. https://doi.org/10.1093/ps/86.5.1050.
- Wang, F., L. Lu, S. Li, S. Liu, L. Zhang, J. Yao, and X. Luo. 2012. “Relative Bioavailability of Mn Proteinate for Broilers Fed a Conventional Corn-Soybean Meal Diet.” Biological Trace Element Research 146 (2): 181–186. https://doi.org/10.1007/s12011-011-9238-z.
- Wang, J., Z. Y. Wang, Z. J. Wang, R. Liu, S. Q. Liu, and L. Wang. 2015. “Effects of Mn Deficiency on Chondrocyte Development in Tibia Growth Plate of Arbor Acres Chicks.” Journal of Bone and Mineral Metabolism 33 (1): 23–29. https://doi.org/10.1007/s00774-014-0563-0.
- Wang, R., Z. Shi, J. Li, D. Tang, S. Qin, and Y. Guo. 2022. “Protective Effect of Mn on Apoptosis and Mitochondrial Function of Heat-Stressed Primary Chick Embryonic Myocardial Cells.” Biological trace element research 200 (10): 4419–4429. https://doi.org/10.1007/s12011-021-03016-2.
- Wołonciej, M., E. Milewska, and W. Roszkowska-Jakimiec. 2016. “Trace Elements As an Activator of Antioxidant Enzymes.” Postepy higieny i medycyny doswiadczalnej (Online) 70 (): 1483–1498. https://doi.org/10.5604/17322693.1229074.
- Xia, W. H., L. Tang, Z. Y. Wang, and L. Wang. 2022. “Effects of Inorganic and Organic Mn Supplementation on Growth Performance, Tibia Development and Oxidative Stress in Broiler Chickens.” Biological Trace Element Research 200 (10): 4453–4464. https://doi.org/10.1007/s12011-021-03041-1.
- Xie, Q., K. Xie, J. Yi, Z. Song, H. Zhang, and X. He. 2022. “The Effects of Magnolol Supplementation on Growth Performance, Meat Quality, Oxidative Capacity and Intestinal Microbiota in Broilers.” Poultry Science 101 (4): 101722. https://doi.org/10.1016/j.psj.2022.101722.
- Yang, T., X. Wang, M. Wen, H. Zhao, G. Liu, X. Chen, G. Tian, J. Cai, and G. Jia. 2021. “Effect of Mn Supplementation on the Carcass Traits, Meat Quality, Intramuscular Fat and Tissue Mn Accumulation of Pekin Duck.” Poultry Science 100 (5): 101064. https://doi.org/10.1016/j.psj.2021.101064.
- Yang, X. J., X. X. Sun, C. Y. Li, X. H. Wu, and J. H. Yao. 2011. “Effects of Copper, Iron, Zinc and Mn Supplementation in a Corn and Soybean Meal Diet on the Growth Performance, Meat Quality and Immune Responses of Broiler Chickens.” Journal of Applied Poultry Research 20 (3): 263–271. https://doi.org/10.3382/japr.2010-00204.
- Zarghi, H., A. Hassanabadi, and N. Barzegar. 2023. “Effect of Organic and Inorganic Mn Supplementation on Performance and Eggshell Quality in Aged Laying Hens.” Veterinary Medicine and Science 9 (3): 1256–1268. https://doi.org/10.1002/vms3.1116.
- Zhang, H., K. Mehmood, X. Jiang, W. Yao, M. Iqbal, K. Li, X. Tong, et al. 2018. “Effect of Icariin on Tibial Dyschondroplasia Incidence and Tibial Characteristics by Regulating P2RX7 in Chickens.” BioMed Research International 2018:1–11. https://doi.org/10.1155/2018/6796271.
- Zhang, J., S. Huang, X. Tong, L. Zhang, X. Jiang, H. Zhang, K. Mehmood, and J. Li. 13 2019. “Chlorogenic Acid Alleviates Thiram-Induced Tibial Dyschondroplasia by Modulating Caspases, BECN1 Expression and ECM Degradation.” International Journal of Molecular Sciences 20 (13): 3160. https://doi.org/10.3390/ijms20133160.
- Zhao, F., C. He, H. Peng, K. Zhang, X. Ding, J. Wang, Q. Zeng, Y. Xuan, S. Bai, and C. Yu. 2019. “Relative Bioavailability of Humate-Mn Complex for Broilers Fed a Corn-Soya Bean Meal Diet.” Journal of Animal Physiology and Animal Nutrition 103 (1): 108–115. https://doi.org/10.1111/jpn.13009.
- Zhaojun, W., W. Lin, W. Zhenyong, W. Jian, and L. Ran. 2013. “Effects of Mn Deficiency on Serum Hormones and Biochemical Markers of Bone Metabolism in Chicks.” Journal of Bone and Mineral Metabolism 31 (3): 285–292. https://doi.org/10.1007/s00774-012-0417-6.
- Zhu, M. J., S. P. Ford, P. W. Nathanielsz, and M. du. 2004. “Effect of Maternal Nutrient Restriction in Sheep on the Development of Fetal Skeletal Muscle.” Biology of Reproduction 71 (6): 1968–1973. https://doi.org/10.1095/biolreprod.104.034561.
- Zofková, I., P. Nemcikova, and P. Matucha. 2013. “Trace Elements and Bone Health.” Clinocal Chemistry and Laboratory Medicine 51 (8): 1555–1561. https://doi.org/10.1515/cclm-2012-0868.