Abstract
Aluminum (Al) toxicity is one of the major abiotic stress factors that decreases crop yield in acidic soils. Al inhibits the root growth and development due to cellular toxicity including nucleus, chromosome and DNA alterations. Although some Al-induced programmed cell death (PCD) features were identified, there are limited comparative and time-dependent studies. The aim of this study is to compare the Al-induced time-dependent DNA fragmentation, the most widely evaluated criterion for PCD in six agronomic plant (maize, wheat, triticale, rye, barley and oat) roots concerning their Al tolerance. Roots were exposed to 100 μM AlCl3 solution (pH 4.5) for different times (½, 1, 2, 3, 4, 5, 6, 7, 8 h). All genomic DNA was isolated from control and AlCl3 treated roots. Isolated DNA samples were analyzed by electrophoretically and DNA fragmentation was observed in triticale, rye, barley and oat roots, but not in maize and wheat. The obtained DNA fragmentation shows that wheat and maize are more tolerant than triticale, rye, barley and oat in which the PCD is active as early as ½ h after Al induction. The results obtained can provide a better understanding into the Al induced PCD and Al tolerance in plants.
Introduction
In natural habitats, plants are frequently exposed to a variety of environmental stresses because of their sessile nature. In excessive stress, agricultural crops and wild flora can be damaged causing considerable environmental and economic losses worldwide (Tuzhikov et al. 2011). In the course of evolution, and also due to targeted selection, many plant species have developed alternative adaptive mechanisms in which the cells and tissues undergo programmed death under extreme abiotic and biotic stresses, to enable the survival of whole organism (Drew et al. Citation2000; Jackson and Armstrong Citation1999).
Programmed cell death (PCD) is a genetically controlled process which originates during development and particularly under biotic–abiotic stress responses (Vardar and Ünal Citation2011a; Wang et al. Citation2011). PCD in plants and animals is associated with cell shrinkage, vacuolization, breakdown of cellular contents, cytochrome c release from mitochondria, specific protease activation (e.g. caspases and metacaspases), chromatin condensation and DNA fragmentation (Qiao et al. Citation2003; Brighigna et al. Citation2006; Vardar and Ünal Citation2008; Papini et al. Citation2011; Wang et al. Citation2012; Poor et al. Citation2013).
Although there are many ultrastructural studies characterizing PCD, DNA fragmentation is the most widely evaluated and sensitive parameter. The fragmentation can be visualized by TUNEL (terminal deoxynucleotidyl transferase dUTP nick end labeling) assay, comet analysis and laddering on the gel due to the internucleosomal cleavage of DNA by specific proteases. In plants, DNA fragmentation has been presented in aleurone layers during seed germination (Wang et al. Citation1998), anther development (Vardar and Ünal Citation2011b;Citation2012), leaf senescence (Yen and Yang Citation1998), in response to pathogens (Wang et al. Citation1996; Navarre and Wolpert Citation1999) and abiotic stress treatment (Danon and Gallois Citation1998).
Aluminum (Al), an abiotic stress factor, is the third most widespread mineral in the earth’s crust, comprising approximately 8% of the crust’s minerals. It is also one of the major plant growth-limiting factors on acid soils (Kochian et al. Citation2004), which cover approximately 67% of the world’s potential arable land (von Uexküll and Mutert Citation1995; Eswaran et al. Citation1997; Abate et al. Citation2013). Al exists as a non-toxic component of soil in the form of insoluble aluminosilicates or oxides, but complex Al becomes soluble when the soil pH is below 5.0 (Matsumoto Citation2000; Vardar and Ünal Citation2007).
The root apex is the primary target of phytotoxic aluminum ions. For most agronomic plants, Al rapidly inhibits root growth at micromolar concentrations (Samac and Tesfaye Citation2003). Al toxicity results in shortened and stunted roots (Vardar et al. Citation2006), leading to poor nutrition absorption, less water uptake and decreased biomass (Kochian Citation1995; Melakeberhan et al. Citation2001). Al sensitive plants have greatly decreased yield and crop quality (Samac and Tesfaye Citation2003). Recent studies have described some cell death features subsequent to Al treatment in plant cells, including DNA fragmentation (Pan et al. Citation2001), changes in nucleus morphology (Panda et al. Citation2008), mitotic abnormalities (Vardar et al. 2011), caspase like activities (Aytürk and Vardar Citation2015), cytochrome c release (Huang et al. Citation2014), and double strand DNA breaks (Vardar et al. 2015) and others have identified the potential roles of anti-apoptotic members in Al tolerance (Yakimova et al. Citation2007; Zheng et al. Citation2007).
Cereals such as maize (Zea mays L.), wheat (Triticum aestivum L.), rye (Secale cereale L.), triticale (Triticosecale wittmack L.), barley (Hordeum vulgare L.) and oat (Avena sativa L.) belonging to Gramineae (Poaceae) have been bred for centuries and are considered essential nutrition for the world’s population, being cultivated on approximately half of the world’s crop land (Bartlett et al. Citation2008). The genetic potential of cereals has been limited due to environmental pollution and climate change. For instance, acid soils covering 67% of arable lands and more than 50% of potential arable lands cause multiple abiotic stresses such as Al toxicity (Ma et al. Citation2014). It has been reported that solubilized aluminum exists in a range of 10–100 μM in acid soils and affects plant growth within a few minutes to a few hours (Ciamporova Citation2002; Barceló and Poschenrieder Citation2002; Vardar and Ünal Citation2007; Abate et al. Citation2013).
Variations in Al tolerance have been identified in many gramineous species and cultivars, and selective breeding programs have produced crop varieties with improved Al tolerance. Characterization of these plants with multiple analyses has led to a better understanding of the mechanisms of Al tolerance. Although there have been multiple morphological, biochemical and molecular studies of Al-induced phytotoxicity, there are limited Al-induced PCD analyses for selection of Al tolerant varieties and development of plants with improved performance in acid soils (Samac and Tesfaye Citation2003).
The aim of this study is to compare the time-dependent acute DNA fragmentation induced by Al under acidic conditions in six agronomic plant roots – maize, wheat, rye, triticale, barley and oat – concerning their Al tolerance.
Materials and methods
Maize (Zea mays L. cv Karadeniz Yıldızı), wheat (Triticum aestivum L. cv Demir 2000), rye (Secale cereale L. cv Aslım 95), triticale (Triticosecale wittmack L. cv Mikham 2002), barley (Hordeum vulgare L. cv Çetin 2000) and oat (Avena sativa L. cv Seydişehir) seeds obtained from Ankara, Black Sea and Bahri Dağdaş Agricultural Research Institutes (Turkey) were sterilized with 1% sodium hypochloride solution for 10 min. Having rinsed with sterile deionized water several times, seeds were placed for germination on moistened filter paper in Petri dishes under plant growth room conditions (temperature of 23 ± 2°C, relative humidity 45–50%, and fluorescent tubes giving an irradiance of 5000 lux, day/night 16/8 respectively). Thirty seeds were used for each experimental group.
Following Pan et al. (Citation2001), germinated seeds which reached 0.5–1 cm root elongation were exposed to 100 μM AlCl3 solution (pH 4.5) at different times (½, 1, 2, 3, 4, 5, 6, 7, 8 h). Deionized water was used for the control group. After AlCl3 treatment, root tips were rinsed with deionized water. Apical root tips (10 mm) excised from 10–12 roots were ground to a fine powder in liquid nitrogen. Genomic DNA was isolated from control and AlCl3 treated roots, according to Hameed et al. (Citation2004). The homogenized roots were incubated in 600 μl extraction buffer (100 mM Tris-HCl pH 8.0, 1.5 M NaCl, 20 mM EDTA, 1% sodium dodecyl sulfate, 0.2% 2-mercaptoethanol) in a hot plate at 65°C for 10 min. Following the incubation 600 μl mixture of chloroform:isoamyl alcohol (24:1 v/v) was added to each sample and gently mixed at room temperature. Samples were centrifuged at 4000 rpm for 5 min and the clear aqueous phases were transferred into new sterile tubes. Two volumes of ice-cold 96% ethanol were added and centrifuged at 10,000 rpm for 10 min. The supernatants were removed and dried in a vacuum concentrator. Pellets were dissolved in 20 μl TE (0.5 mM EDTA pH 8.0, 10 mM Tris-HCl) buffer. Contaminating RNA was removed by incubating at 37°C for 25 min in the presence of DNase-free ribonuclease A (50 μg ml−1). After addition of three volumes of ice-cold 96% ethanol, the samples were centrifuged at 10,000 rpm for 10 min to pellet DNA. The supernatants were removed carefully and pellets were dried. Genomic DNA was dissolved in 40 μl TE buffer and stored at –20°C for further use. Quantity of DNA was measured with a Qubit fluorometer, Invitrogen, USA using Qubit dsDNA assay kits. DNA samples (50 ng μl−1) were run on a 1.5% (w/v) agarose gel at 100 V for 2 h. The genomic DNA was stained with red safe (1/20,000) and analyzed under a Genoplex UV transilluminator, VWR, USA. All the analyses were repeated with three replicates.
Results and discussion
We aimed to characterize the time-dependent occurrence of Al-induced DNA fragmentation in six different Gramineae species. Based on electrophoretic analysis, no DNA fragmentation was observed in roots of maize (Figure ) and wheat (Figure ) up to 8 h. DNA ladders were observed as early as ½ h in triticale (Figure ), and were visible slightly after ½ h and significantly after 1 h in rye (Figure ). DNA fragmentation was clear after 1 h in barley (Figure ); the fragmentation was slight after 1 h in oat and became distinctive after 2 h (Figure ). There was no change in DNA fragmentation profile up to 8 h. DNA ladder occurrence, indicating that oligonucleosomal fragments of DNA were cleaved by special endonucleases in the linker regions between nucleosomal cores, suggests that wheat and maize are more tolerant than triticale, rye, barley and oat in which the PCD is active as early as ½ h after Al induction.
Figure 1. Analyses of agarose gel electrophoresis on root genomic DNA of Zea mays (maize). No DNA fragmentation occurred. M, 100 bp marker; C, control; h, hour.
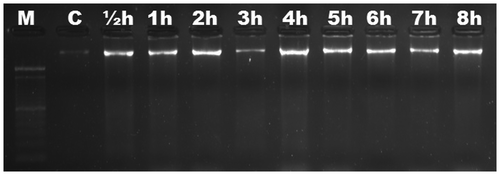
Figure 2. Analyses of agarose gel electrophoresis on root genomic DNA of Triticum aestivum (wheat). No DNA fragmentation occurred. M, 100 bp marker; C, control; h, hour.
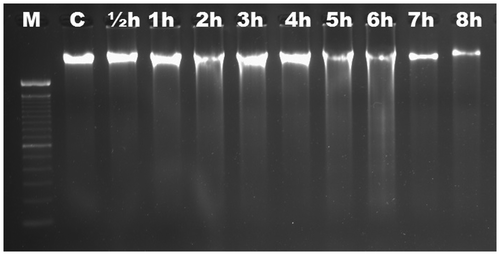
Figure 3. Analyses of agarose gel electrophoresis on root genomic DNA of Triticosecale wittmack (triticale). DNA fragmentation occurred at ½ h. M, 100 bp marker; C, control; h, hour.
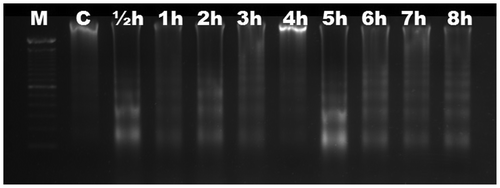
Figure 4. Analyses of agarose gel electrophoresis on root genomic DNA of Secale cereale (rye). DNA fragmentation occurred slightly at ½ h (arrows). M, 100 bp marker; C, control; h, hour.
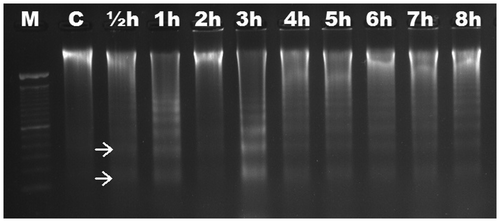
Figure 5. Analyses of agarose gel electrophoresis on root genomic DNA of Hordeum vulgare (barley). DNA fragmentation occurred at 1 h. M, 100 bp marker; C, control; h, hour.
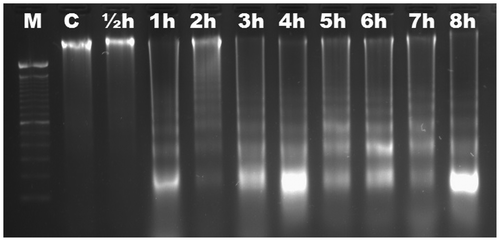
Figure 6. Analyses of agarose gel electrophoresis on root genomic DNA of Avena sativa (oat). DNA fragmentation occurred slightly at 1 h (arrows). M, 100 bp marker; C, control; h, hour.
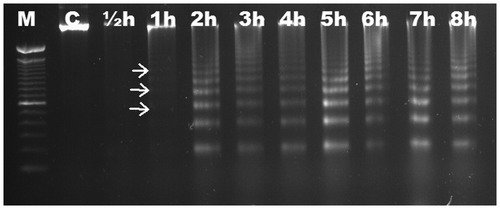
Aluminum has toxic effects on the cell wall, plasma membrane, cytoskeleton and nucleus, which are among the most important Al binding sites (Matsumoto Citation2000). Although Al phytotoxicity has been investigated intensively and extensively, the mechanism of Al toxicity and tolerance remains to be clarified. Recently more attention has been paid to Al-induced PCD effects, including mitochondrial dysfunction (Li and Xing Citation2011), caspase like activities (Aytürk and Ünal 2015), nucleus morphology changes (Pan et al. Citation2001) and appearance of nucleus/DNA fragmentation (Huang et al. Citation2014) in plant cells.
In an early study evaluating Al-induced DNA fragmentation, Pan et al. (Citation2001) treated Hordeum vulgare L. roots with Al in a range of 0.1–50 mM. The researchers detected clear DNA ladders in Al concentrations of 0.1–1 mM at 8 h. However, a necrotic DNA smear was observed at 10–50 mM. Achary et al. (Citation2008) used the comet assay to show that Al induced DNA damage in a range of 50–200 μM AlCl3 after 2 h in Allium cepa roots; this assay detects breaks in double stranded DNA in relation to the induction of PCD. A similar process was performed by Achary and Panda (Citation2009) with higher concentrations of Al (200, 400 and 800 μM) for 3 h in A. cepa and Al induced DNA damage was evaluated. Subsequently Achary et al. (Citation2012) treated the H. vulgare roots with different concentrations of Al (2.5, 5 and 10 mM) for 12 h and Al induced DNA damage was observed in a dose-dependent manner. Moreover, Huang et al. (Citation2014) treated Arachis hypogaea (peanut) roots with 100 μM AlCl3 and detected DNA fragmentation after 4 h. The significance of the present study is that it is the first to compare time-dependent DNA fragmentation in the roots of six Gramineae species, and to provide the impact time of Al toxicity on genomic DNA.
One of the common responses to Al toxicity is the increase of ROS (reactive oxygen species) generation (Yamamato et al. Citation2001) causing cellular toxicity (Li and Xing Citation2010). ROS burst subsequently induced the opening of the mitochondrial permeability transition pore, reduced the potential of the inner mitochondrial membrane, causing release of cytochrome c into the cytosol and the activation of caspase-3-like protease, which resulted in the execution of PCD (Li and Xing Citation2010; Huang et al. Citation2014; Petrov et al. Citation2015).
Caspases (cysteine-dependent aspartate-specific proteases) are a family of cysteine proteases that play essential roles in PCD. They are responsible for initiating PCD, executing PCD and PCD signal transduction in animals. Although animals have caspase genes, plants do not have orthologous sequences in their genomes. The caspase-like activities have been indicated in plants by the application of artificial synthesized substrates and inhibitors of caspase (Wang et al. Citation2010; Li and Xing Citation2011; Poor et al. Citation2013). Among various kinds of animal caspases, caspase-3, responsible for DNA fragmentation and chromatin condensation, is considered to be the major executioner in PCD (Zhang et al. Citation2009). Huang et al. (Citation2014) reported both DNA fragmentation and caspase-3 like activity after 100 μM AlCl3 treatment in A. hypogaea root tips. Aytürk and Vardar (Citation2015) demonstrated caspase 3, 8 and 9 like activities in rye, triticale, barley and oat within 8 h. Then, comparison of Al tolerance is as follows: wheat=maize > oat > barley > rye > triticale.
In conclusion, if we rank our tested species according to first DNA fragmentation time, maize and wheat are the most Al tolerant species, showing no DNA laddering at 8 h. Then, comparison of Al tolerance is as follows: oat > barley > rye > triticale.
Genomic stability and protection are essential to sustain survival and biodiversity of the species (Achary and Panda Citation2009). Particularly Al induced PCD responses should be of considerable interest, not only from the principal perspective but also in the content of applied agriculture, environmental protection and ecology (Samac and Tesfaye Citation2003; Tuzhikov et al. Citation2011). Physiological and molecular characterization of Al tolerant plants will lead in the future to a better understanding of the mechanisms of Al toxicity and tolerance during the production of selective breeding programs.
Acknowledgements
We express our gratitude to Prof. Dr Nermin Gözükırmızı (Department of Molecular Biology and Genetics, İstanbul University) for guiding us towards Al toxicity studies and Prof. Dr Meral Ünal (Department of Biology, Marmara University) for guiding us towards PCD studies.
Additional information
Funding
References
- Abate E, Hussien S, Laing M, Mengistu F. 2013. Aluminum toxicity tolerance in cereals: Mechanisms, genetic control and breeding methods. Afr J Agric Res. 8(9):711–722.
- Achary VMM, Jena S, Panda KK, Panda BB. 2008. Aluminium induced oxidative stress and DNA damage in root cells of Allium cepa L. Ecotoxicol Environ Saf. 70(2):300–310.
- Achary VMM, Panda BB. 2009. Aluminium-induced DNA damage and adaptive response to genotoxic stress in plant cells are mediated through reactive oxygen intermediates. Mutagenesis. 25(2):201–209.
- Achary VMM, Patnaik AR, Panda BB. 2012. Oxidative biomarkers in leaf tissue of barley seedlings in response to aluminium stress. Ecotox Environ Safe. 75(1):16–26.
- Aytürk Ö, Vardar F. 2015. Aluminum induced caspase-like activities in some Gramineae species. Adv Food Sci. 37(2):71–75.
- Barceló J, Poschenrieder C. 2002. Fast root growth responses, root exudates and internal detoxification as clues to the mechanisms of aluminium toxicity and resistance: a review. Environ Exp Bot. 48(1):75–92.
- Bartlett JG, Alves SC, Smedley M, Snape JW, Harwood WA. 2008. High-throughput Agrobacterium-mediated barley transformation. Plant Methods. 4(1):1–12.
- Brighigna L, Milocani E, Papini A, Vesprini JL. 2006. Programmed cell death in the nucellus of Tillandsia (Bromeliaceae). Caryologia. 59(4):334–339.
- Ciamporova M. 2002. Morphological and structural responses of plant roots to aluminum at organ, tissue and cellular levels. Biol Plant. 45(2):161–171.
- Danon A, Gallois P. 1998. UV-C radiation induces apoptotic-like changes in Arabidopsis thaliana. FEBS Lett. 437(1–2):131–136.
- Drew MC, He CJ, Morgan PW. 2000. Programmed cell death and aerenchyma formation in roots. Trends Plant Sci. 5(3):123–127.
- Eswaran H, Reich P, Beinroth F. 1997. Global distribution of soils with acidity. In: Moniz ACMF, Schaffert RE, Fageria NK, Rosolem CA, Cantarella H, editors. Plant–soil interactions at low pH. Brazil: Brazilian Soil Science Society. p. 159–164.
- Hameed A, Malik SA, İqbal N, Arshad R, Farooq S. 2004. A rapid (100 min) method for isolating high yield and quality DNA from leaves, roots and coleoptile of wheat (Triticum aestivum L.) suitable for apoptotic and other molecular studies. Int J Agric Biol. 2(2):383–387.
- Huang WJ, Oo TL, He HY, Wang AQ, Zhan J, Li CZ, Wei SQ, He LF. 2014. Aluminum induced rapidly mitochondria dependent programmed cell death in Al sensitive peanut root tips. Bot Stud. 55(1):67–78.
- Jackson MB, Armstrong W. 1999. Formation of aerenchyma and the processes of plant ventilation in relation to soil flooding and submergence. Plant Biol. 1(3):274–287.
- Kochian LV. 1995. Cellular mechanism of aluminium toxicity and resistance in plants. Annu Rev Plant Physiol Plant Mol Biol. 46:237–260.
- Kochian LV, Hoekenga OA, Pineros MA. 2004. How do crop plants tolerate acid soils? Mechanisms of aluminum tolerance and phosphorous efficiency. Annu Rev Plant Biol. 55:459–493.
- Li Z, Xing D. 2010. Mitochondrial pathway leading to programmed cell death induced by aluminum phytotoxicity in Arabidopsis. Plant Signal Behav. 5(12):1660–1662.
- Li Z, Xing D. 2011. Mechanistic study of mitochondria dependent programmed cell death induced by aluminum phytotoxicity using fluorescence techniques. J Exp Bot. 62(1):331–343.
- Ma JF, Chen ZC, Shen RF. 2014. Molecular mechanism of Al tolerance in gramineous plants. Plant Soil. 381:1–12.
- Matsumoto H. 2000. Cell biology of aluminum toxicity and tolerance in higher plants. Int Rev Cytol. 200:1–47.
- Melakeberhan H, Bird GW, Jones AL. 2001. Soil pH affects nutrient balance in cherry rootstock leaves. Hort Science. 36:916–917.
- Navarre DA, Wolpert TJ. 1999. Victorin induction of an apoptotic/senescence-like response in oats. Plant Cell. 11(5):237–249.
- Pan JW, Zhu MY, Chen H. 2001. Aluminum-induced cell death in root-tip cells of barley. Environ Exp Bot. 46(2):71–79.
- Panda SK, Yamamoto Y, Kondo H, Matsumoto H. 2008. Mitochondrial alterations related to programmed cell death in tobacco cells under aluminium stress. C R Biol. 311(2):597–610.
- Papini A, Mosti S, Milocani E, Tani G, Di Falco P, Brighigna L. 2011. Megasporogenesis and programmed cell death in Tillandsia (Bromeliaceae). Protoplasma. 248(8):651–662.
- Petrov V, Hille J, Mueller-Roeber B, Gechev TS. 2015. ROS-mediated abiotic stress-induced programmed cell death in plants. Front Plant Sci. 6(4):69.
- Poor P, Kovacs J, Szopko D, Tari I. 2013. Ethylene signaling in salt stress- and salicylic acid-induced programmed cell death in tomato suspension cells. Protoplasma. 250:273–284.
- Qiao JJ, Yuan YJ, Zhao H, Wu JC. 2003. Differences in protein patterns in suspension cultures of Taxus cuspidata induced by cerium. Biol Plant. 46(1):611–615.
- Samac DA, Tesfaye M. 2003. Plant improvement for tolerance to aluminum in acid soils – a review. Plant Cell Tiss Org. 75(4):189–207.
- Tuzhikov AI, Vartapetian BB, Vartapetian AB, Chichkova NV. 2011. Abiotic stress-induced programmed cell death in plants: A phytaspase connection. In: Shanker A, editor. Abiotic stress responses in plants – Physiological, biochemical and genetic perspectives. Croatica: Intech Croatica. p. 183–196.
- Vardar F, Akgül N, Aytürk Ö, Aydın Y. 2015. Assessment of aluminum induced genotoxicity with comet assay in wheat, rye and triticale roots. Fresen Environ Bull. 37(10a):3352–3358.
- Vardar F, Arıcan E, Gözükırmızı N. 2006. Effects of aluminum on in vitro root growth and seed germination of tobacco (Nicotiana tabacum L.). Adv Food Sci. 28(2):85–88.
- Vardar F, İsmailoğlu I, İnan D, Ünal M. 2011. Determination of stress responses induced by aluminum in maize (Zea mays L. Karadeniz Yıldızı). Acta Biol Hung. 62(2):156–170.
- Vardar F, Ünal M. 2007. Aluminum toxicity and resistance in higher plants. Adv Mol Biol. 1(1):1–12.
- Vardar F, Ünal M. 2008. Proteolytic enzymes in plant programmed cell death. Turk J Sci Rev. 1(1):65–78.
- Vardar F, Ünal M. 2011a. Immunolocalization of lipoxygenase in the anther wall cells of Lathyrus undulatus Boiss. during programmed cell death. Not Bot Hort Agrobo. 39(1):71–78.
- Vardar F, Ünal M. 2011b. Development and programmed cell death in the filament cells of Lathyrus undulatus Boiss. Caryologia. 64(2):164–172.
- Vardar F, Ünal M. 2012. Ultrastructural aspects and programmed cell death in the tapetal cells of Lathyrus undulatus Boiss. Acta Biol Hung. 63(1):56–70.
- von Uexküll HR, Mutert E. 1995. Global extent, development and economic impact of acid soils. Plant Soil. 171:1–15.
- Wang EJ, Li X, Liu Y, Zhao X. 2010. Salt stress induces programmed cell death in Thellungiella halophile suspension-cultured cells. J Plant Physiol. 167(14):1145–1151.
- Wang GP, Zhang ZH, Kong DJ, Liu QX, Zhao GL. 2012. Programmed cell death is responsible for replaceable bud senescence in chestnut (Castanea mollissima BL.). Plant Cell Rep. 31(9):1603–1610.
- Wang H, Li J, Bostock RM, Gilchrist DG. 1996. Apoptosis: a functional paradigm for programmed plant cell death induced by a host-selective phytotoxin and invoked during development. Plant Cell. 8(3):375–391.
- Wang J, Li X, Liu Y, Zhao X, Chen C, Tian F. 2011. MEK/ERK inhibitor U0126 enhanced salt stress-induced programmed cell death in Thellungiella halophila suspension-cultured cells. Plant Growth Regul. 63:207–216.
- Wang M, Oppedijk BJ, Caspers MPM, Lamers GEM, Boot MJ, Geerlings DNG, Bakhuizen B, Meijer AH, van Duijn B. 1998. Spatial and temporal regulation of DNA fragmentation in the aleurone of germinating barley. J Exp Bot. 49(325):1293–1301.
- Yakimova ET, Kapchina-Toteva VM, Woltering EJ. 2007. Signal transduction events in aluminium-induced cell death in tomato suspension cells. J Plant Physiol. 164(6):702–708.
- Yamamato Y, Kobayashi Y, Matsumoto H. 2001. Lipid peroxidation is an early symptom triggered by aluminum, but not the primary cause of elongation inhibition in pea root. Plant Physiol. 125(1):199–208.
- Yen CH, Yang CH. 1998. Evidence for programmed cell death during leaf senescence in plants. Plant Cell Physiol. 39(9):922–927.
- Zhang L, Xu Q, Xing D, Gao C, Xiong H. 2009. Real time detection of caspase-3 like protease activation in vivo using fluorescence resonance energy transfer during plant programmed cell death induced by ultraviolet C overexposure. Plant Physiol. 150(4):1773–1783.
- Zheng K, Pan JW, Ye L, Fu Y, Peng HZ, Wan BY, Gu Q, Bian HW, Han N, Wang JH, Kang B, Pan JH, Shao HH, Wang WZ, Zhu MY. 2007. Programmed cell death-involved aluminium toxicity in yeast alleviated by antiapoptotic members with decreased calcium signals. Plant Physiol. 143(1):38–49.