Abstract
In vitro and in vivo experiments on human cell lines (breast adenocarcinoma, MCF-7, and liver hepatocellular carcinoma, HepG2), plants (Allium cepa) and bacteria (Staphylococcus aureus and Pseudomonas aeruginosa) displayed cytotoxic and genotoxic impacts of silver nanoparticles (AgNPs). The cell viability assays indicated that AgNPs had inhibitory effects on MCF-7 cell line more effectively than HepG2 cell line. Mitotic indices and distribution of cells in mitotic phases of A. cepa were clearly changed after treatment with three different weight ratios of silver metal (1, 3 and 5%) for 3 and 12 h. With increasing concentration of the nanoparticles a decrease in the mitotic index was detected (from 4.19 to 0.79 after 3 h and from 4.56 to 0.25 after 12 h) and different types of chromosomal aberrations were noticed. Protein electrophoresis results of S. aureus and P. aeruginosa demonstrated the change in the protein profiles of the two bacterial strains and it was more noticeable in P. aeruginosa than S. aureus. RAPD-PCR and ISSR-PCR analyses using different primers (OP-A19, OP-B04, OP-C04, OP-C12, OP-Q18, OP-Z03, 14A, 44B, HB-08, HB-11 and HB-12) demonstrated the impairment of bacterial DNA and induction of genetic variability. The percentage of polymorphism was higher in P. aeruginosa than S. aureus. The present findings revealed an analogous effect of AgNPs induced in in vitro and in vivo experiments, therefore AgNP applications should be given special consideration.
1. Introduction
Nanoscience is a new interdisciplinary science with considerable influence on economy, society and environment. Nanomaterials are defined by their small size (< 100 nm) and their novel physical and chemical properties, which are progressively used on different research and economical fields. However, the rapid progress in nanoscience has not been accompanied by enough information regarding its hazards. Previous studies showed that exposure to nanoparticles could cause several diseases like inflammation, oxidative stress, myocardial infarction and thrombosis (Kumar Citation2006). The large surface area and small size of nanoparticles are the main factors of their free translocation when inhaled, causing toxicity (Oberdörster Citation1996; Abdel-Azeem and Elsayed Citation2013). Although these are general perceptions, each nanoparticle should need exhaustive study for usage.
Silver metal (Ag) is used for the synthesis of silver nanoparticles (AgNPs) (Ahmad et al. Citation2003). Silver ions are categorized by the Environmental Protection Agency (EPA) as an environmental hazard because silver is the most toxic element after mercury to plants and animals. The unique properties of AgNPs make them ideal for numerous technologies, including biomedical, optical materials, optical, and antimicrobial applications (Kim et al. Citation2007; Choi et al. Citation2008). AgNPs are widely used in medical purposes such as treating wounds, burns and catheter related infections (Samuel and Guggenbichler Citation2004). AgNPs are classified as antimicrobial agents (Elechiguerra et al. Citation2005; Shahverdi et al. Citation2007), as they can change the bacterial membrane structure by binding to the sulphur-containing proteins of the cell membrane, causing bacterial death (Sondi and Salopek-Sondi Citation2004; Kheiralla et al. Citation2014).
Pulate et al. (Citation2011) and CitationPatlolla et al. (2012) reported the cytotoxic and genotoxic impact of AgNPs on A. cepa and V. faba meristems. Even if a nanoparticle itself is not toxic, AgNPs increase the effectiveness of delivering toxic silver ions to locations where they can cause toxicity (AshaRani et al. Citation2009). Toxicity of AgNPs has been studied in different cellular models such as the germ line stem cells (Braydich-Stolle et al. Citation2005) and human lung fibroblasts (AshaRani et al. Citation2009). Also, AgNPs have been shown to be genotoxic in plant cells (Kumari et al. Citation2009; Vannini et al. Citation2014). Proteomic studies on Arabidopsis thaliana and Eruca sativa partially declared the toxic response of previous plants to AgNPs and Ag+ (Kaveh et al. Citation2013; Vannini et al. Citation2013).
Metal ions including silver (Ag) act as genotoxic agents by producing reactive oxygen species (ROS) (Carlson et al. Citation2008), which can induce oxidative DNA damage (Hussain et al. Citation2005; Kumari et al. Citation2011; Vannini et al. Citation2014). The oxidative stress and the generation of ROS by AgNPs was demonstrated by several studies in unicellular algae Chlamydomonus reinhardtii (Navarro et al. Citation2008), rat alveolar macrophages (Carlson et al. Citation2008), and human hepatoma cells (Kim et al. Citation2009).
The hazards associated with nanoparticle usage and the molecular response underlying AgNP toxicity are still completely unknown. In this study we aimed to explore the genotoxic and DNA-damaging potential of manufactured AgNPs by two different genotoxicity tests using both in vitro and in vivo systems using human cell lines and the well-established A. cepa root bioassay. The Allium assay is a fast and sensitive assay used extensively for detection of environmental genotoxins and mutagens. Leme and Marine-Morales (Citation2009) reported that the A. cepa test is used in environmental monitoring of chemicals and provides a high degree of correlation with the genotoxicity assays of both human and mammalian. The human cell lines are used in a cytotoxicity assay to determine the IC50 of AgNPs to be used for medical purposes. Furthermore, the present study evaluates the potential of AgNPs to induce genetic variations in a two bacterial model systems (S. aureus and P. aeruginosa). These bacteria are used as a biological system for molecular biology studies. Genetic variations were evaluated using two molecular marker techniques: random amplified polymorphic DNA (RAPD), and inter simple sequences repeat (ISSR). For biochemical analysis, the sodium dodecyl sulphate polyacrylamide gel electrophoresis (SDS-PAGE) molecular approach was used to assess genetic variation, which may help to better understand the harmful effects of AgNPs on the environment and living beings.
2. Materials and methods
2.1 Nanoparticles
AgNPs were prepared using the method described previously by Kheiralla et al. (Citation2014). The characterization of nanoparticles was also published in Kheiralla et al. (Citation2014). Briefly, the silver was impregnated on montmorillonite clay (MMT). Clay is used as a drug carrier for several pharmaceutical purposes due to its eco-friendly nature; it is a non-toxic, non-corrosive and recyclable material (Theng Citation1974). The obtained AgNP materials were labelled as 1, 3 and 5% AgNP, where 1, 3 and 5 represented the weight ratio of silver metal and MMT.
2.2 Test system
Two human carcinoma cell lines, MCF-7 (breast adenocarcinoma) and HepG2 (liver hepatocellular carcinoma) were obtained from the VACSERA Tissue Culture Unit (The Egyptian Company for Biotech Industries, Giza, Egypt) and cultured in either RPMI-1640 or DMEM media supplemented with 10% heat-inactivated fetal bovine serum (FBS), 1% L-glutamine, HEPES buffer and 50 μg ml–1 gentamycin. All cells were maintained at 37°C in a humidified atmosphere with 5% CO2 and sub-cultured two times a week. Onions bulbs (Allium cepa L., 2n = 16, variety Giza 6 Mohassan) were obtained from The Agriculture Research Center Giza, Egypt. Bacterial strains (Staphylococcus aureus and Pseudomonas aeruginosa) were isolated from hospitalized patients with indwelling catheter suffering from urinary tract infections (UTIs) by applying the standard methods of Harley and Prescott (Citation2002). Bacteria were cultured in Luria-Bertaini (LB) broth. The bacterial suspension was prepared and the bacterial concentration was adjusted to 1.5 × 108 CFU ml–1 (corresponding to 0.5 McFarland standards).
2.3 Cytotoxicity analysis
2.3.1 Evaluation of in vitro cytotoxic activity
The in vitro cytotoxic activity evaluation of the synthesized AgNPs (1, 3 and 5%) was carried out in two human cancer cell lines, MCF-7 and HepG2, and the cytotoxic effect assay was used according to the procedure adapted by the Regional Center of Mycology and Biotechnology, Egypt. The cells were seeded in 96-well plates at a cell concentration of 1 × 104 cells per well in 100 μμl of growth medium. After incubation of the cells in a humidified incubator with 5% CO2 for a period of 24 h at 37°C, serial dilutions 10, 5, 2.5 and 1 μg of the tested compound (AgNPs@MMT) were added to confluent cell mono layers dispensed into 96-well, flat-bottomed microtitre plates (Falcon, Albany, NY, USA). The microtitre plates were incubated for 48 h. Non-treated cells were used as control. Viable cell yields were determined by a colorimetric method. After the end of the incubation period, media were aspirated and the crystal violet solution (1%) was added to each well for at least 30 min. The stain was removed and the plates were rinsed using tap water until all the excess of stain was removed. Glacial acetic acid (30%) was then added to all wells and mixed thoroughly, then the absorbance of the plates were measured after gently shaken on Microplate reader (TECAN, Inc., Trading AG, Switzerland), using a test wavelength of 490 nm. Treated samples were compared with the control cell in the absence of the tested compounds. All experiments were carried out in triplicate. The cell cytotoxic effect of each tested compound was calculated (Mosmann Citation1983).
2.3.2 Onion root-tip cytotoxic potential test
Forty healthy A. cepa bulbs were used, according to Fiskesjo (Citation1997). The bulbs were soaked in distilled water for 48 h at room temperature in clean vials until the roots reached 3–4 cm in length, then 30 bulbs were transferred to new vials containing different weight ratios of silver metal in AgNPs (1, 3 and 5%) for 3 and 12 h, while 10 bulbs remained in distilled water to be used as control. After treatment, roots were cut and fixed in Carnoy’s solution for 24 h, hydrolysed with 1 N HCl at 60°C for 5 min, washed with distilled water and stained using Schiff’s stain for 1 h in the dark. The meristematic region were carefully squashed in 45% acetic acid then examined at 400× magnification using an Olympus Microscope (BX50, Olympus Company, Tokyo, Japan). Replicates of five bulbs were analysed for each treatment and control. Cytotoxic potential was determined by means of the mitotic index (MI). Mitotic index was defined as the number of dividing cells per 1000 cells. All cells with different types of aberrations were scored and the most representative ones for each abnormality were photographed.
2.3.3 Micronucleus (MN) assay (mutagenicity test)
Forty A. cepa bulbs were soaked in distilled water for 48 h at room temperature in clean vials until the roots reached 3–4 cm in length, then 30 bulbs were transferred to new vials containing different weight ratios of silver metal in AgNPs (1, 3 and 5%) for 3 and 12 h, while 10 bulbs remained in distilled water to be used as control. After treatment, roots were cut, fixed in Carnoy’s solution, hydrolysed with 1 N HCl at 60°C for 5 min, and stained using Schiff’s stain for 1 h in the dark as described above. The root tip regions were carefully squashed in 45% acetic and examined at 400× magnifications using an Olympus Microscope (BX50). Replicates of five bulbs were analysed for each treatment and control. The percentage of micronucleus was scored by counting the number of cells containing micronucleus per 1000 cells according to Ma et al. (Citation1995).
2.4 Biochemical analysis
2.4.1 Electrophoretic detection of bacterial protein by sodium dodecyl sulphate polyacrylamide gel electrophoresis (SDS-PAGE)
The protein profile of control and (1, 3 and 5%) AgNP-treated S. aureus and P. aeruginosa bacterial cells was determined according to Laemmili (Citation1970) with slight modifications (the volume of TEMED was reduced from 30 μl to 20 μl and also the volume of APS was reduced from 1.5 ml to 1.3 ml). 1 ml of each sample was pipetted into Eppendorf tubes and centrifuged at 5000 rpm for 10 min. The bacterial pellet of each treatment was ground in liquid nitrogen. The powdered samples were transferred to a 1.5 ml Eppendorf tube, and the volume brought to 200 μl with extraction buffer (50 mM Tris-HCl, pH 6.8, glycerol 10% w/v, ascorbic acid 0.1%, cysteine hydrochloride 0.1 w/v). Centrifugation at 18,000 rpm for 30 min was carried out to remove debris. Twenty μl of protein sample mixed with loading buffer were applied to 11% SDS-PAGE at 30 mA for 3 h. The gel was stained with silver nitrate stain according to Sammons et al. (Citation1981). The gel was photographed and scanned for analysis using Gene Tools syngene ver. 4.00(a), (Syngene, Cambridge, UK) gel documentation system software.
2.5 Molecular analysis
2.5.1 DNA isolation procedure
Bacterial DNA of control and (1, 3 and 5 %) AgNP-treated S. aureus and P. aeruginosa was extracted using DNeasy Bacteria Mini Kit (Bio Basic, Toronto, Canada) containing AP1, AP2 AP3, AW AE and TBE buffers. Isolation protocol of DNA was according to Ramachandran et al. (Citation2009). DNA samples were stored at –70°C for RAPD and ISSR analyses.
2.5.2 RAPD-PCR analysis
In order to obtain clear reproducible amplification products, different factors were optimized. These factors included PCR temperature cycle profile and concentration of each of the template DNA, primer, MgCl2 and Taq polymerase. A total of 21 random DNA oligonucleotide primers synthesized by Operon Biotechnologies, Inc. (Ebersberg, Germany) were independently used according to Williams et al. (Citation1990) in the PCR reaction. Only six primers succeeded to generate reproducible polymorphic DNA products (OP-A19, OP-B04, OP-C04, OP-C12, OP-Q18 and OP-ZO3). The PCR amplification was performed in a 25 μl reaction volume containing the following: 2.5 μl of dNTPs (2.5 mM), 1.5 μl of Mg Cl2 (25 mM), 2.5 μl of 10× buffer, 2.0 μl of primer (2.5 μM), 2.0 μl of template DNA (50 ng μl–1), 0.3 μl of Taq polymerase (5 U μl–1μ) and 14.7 μl of sterile ddH2O. The reaction mixtures were overlaid with a drop of light mineral oil per sample. Amplification was carried out in a Techni TC-512 PCR, Stafford, UK system. The reaction was subjected to one cycle at 95°C for 5 min, followed by 35 cycles at 94°C for 30 s, 37°C for 30 s, and 72°C for 30 s, then a final cycle of 72°C for 12 min. PCR products were run at 100 V for 1 h on 1.4% agarose gels to detect polymorphism between bacterial strains under study. After electrophoresis, the RAPD patterns were visualized with UV transilluminator. RAPD markers were scored from the gels as DNA fragments present or absent in all lanes. Gels were photographed using a Polaroid camera.
2.5.3 ISSR-PCR analysis
ISSR-PCR analysis was accomplished following Zietkiewicz et al. (Citation1994). Five primers succeeded in generating reproducible polymorphic DNA products (14A, 44B, HB-08, HB-11and HB-12); these were primers synthesized by Operon Biotechnologies, Inc. The PCR amplification was performed as described above. After electrophoresis, the ISSR patterns were visualized with UV transilluminator. ISSR markers were scored from the gels as DNA fragments present or absent in all lanes. Gels were photographed using a Polaroid camera.
The DNA bands generated by each primer in both RAPD and ISSR-PCR analyses were counted and their molecular sizes were compared with those of the DNA markers. The bands scored from DNA profiles generated by each primer were pooled together. Then the presence or absence of each DNA band was treated as a binary character in a data matrix (coded 1 and 0, respectively) to calculate genetic similarity among the studied bacterial strains. Calculation was achieved using Dice similarity coefficients (Dice Citation1945) as implemented in the computer program SPSS-10 (www.nyu.edu/acf/pubs/SPSS_Win/SPSSwindoc_ToC.html). Dendrogram trees were constructed from the results using the unweighted pair group method (UPGMA).
2.6. Statistical analysis
Statistical analysis was carried out using GraphPad (Version 4.0; San Diego, USA, www.graphpad.com) with five replicates for each group, except for the in vitro cytotoxic test, where experiments were carried out in triplicate. Data were expressed as mean ± standard deviation (SD) calculated by one way analysis of variance (ANOVA) and SPSS version 20. The significance was analysed using Tukey’s and Dunnett’s tests (significance was accepted at p ≤ 0.05 and p ≤ 0.01).
3. Results
3.1 Cytotoxic results
The cytotoxic activity of AgNPs with weight ratio of silver metal (1, 3 and 5%) was evaluated against MCF-7 and HepG2 cell lines. Figures and reveal that 5% of AgNPs introduced cytotoxic activities toward tumour cell lines (MCF7 and HEP2); 28.97% of MCF7 tumour cells survived and 37.82% of HepG2 (IC50 = 2.6 and 4.7 respectively). However mild activities were detected in case of 1% and 3% of MCF7 (38.98% and 25.48% of cells survived respectively, IC50 = 8.3 and 4.15 respectively). Negligible effects were observed for the HepG2 cell lines with 1%. The cell viability assays indicated that all concentrations had inhibitory effects on the growth of MCF-7 cell line more effectively than HepG2 cell line.
Figure 1. Cytotoxic activity of three concentrations of silver nanoparticles (μg ml–1) from different weight ratios of silver metal (1, 3 and 5%) against MCF-7 cell line (survival %).
**Statistically significant at p ≤ 0.01.
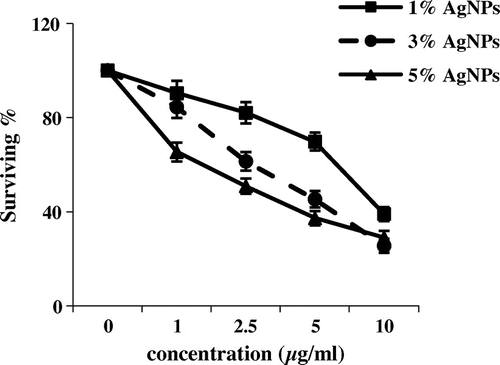
Figure 2. Cytotoxic activity of three concentrations of silver nanoparticles (μg ml–1) from different weight ratios of silver metal (1, 3 and 5%) against HepG2 cell line (survival %).
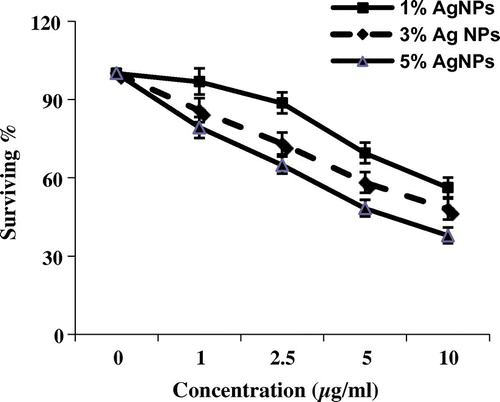
The AgNP suspension had an obvious effect on mitotic cell division and chromosome performance of A. cepa, as can be seen in Figure . The effect of the AgNP suspension was concentration and duration dependent. There was a gradual decrease in the mitotic index (MI) value. Mitotic index was reduced to 0.25% at 5% AgNP suspension for 12 h, which was the lowest value scored. The effect of AgNP suspension on mitotic index was significantly different (p ≤ 0.05, p ≤ 0.01) for 1, 3 and 5% as compared to the control. The highly accumulation of cells at prophase stage was noticed in Table , while the percentage of the rest phases was fluctuated by time and/or concentration of AgNP suspension. The maximum accumulation of prophase was 65.2 at 5% AgNP suspension for 12 h.
Figure 3. Mitotic index of Allium cepa meristems treated with silver nanoparticles. aStatistically significant at p ≤ 0.01.
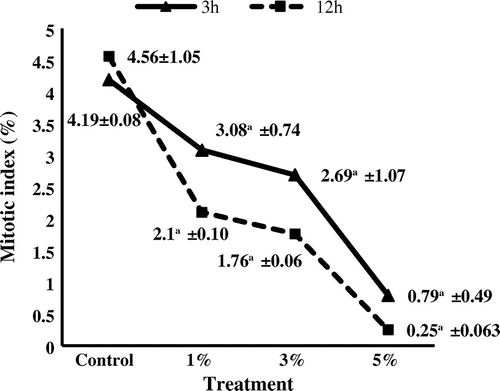
Different chromosomal aberrations were observed: irregular prophase, disturbance, stickiness, break and bridge (Table ). Representative aberrations are illustrated in Figure (a–i).
Figure 4. Types of chromosomal aberrations induced in Allium cepa meristems treated with silver nanoparticles. (a, b) irregular prophase; (c, d) c-metaphase; (e, f) disturbed anaphase; (g) sticky anaphase; (h) chromatin bridge; (i) chromosomal break and micronucleus in metaphase; (j) micronuclei in interphase; (k) uniucleate cell with two micronuclei (binucleate); (l) multinucleate cell in interphase.
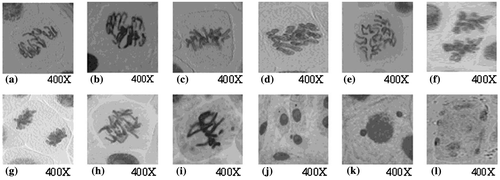
The micronucleus test revealed the genotoxicity of AgNP suspension. The study of DNA damage at the chromosome level is an essential part of genetic toxicology. Micronucleus assays have emerged as one of the preferred methods for assessing chromosome damage because they enable both chromosome loss and chromosome breakage to be measured reliably. The frequencies of induced micronuclei were statistically correlated to AgNP suspension, which followed a dose-response curve. The highest frequency (10.48) was scored after the highest concentration (5%) for 12 h (Figure ). Micronuclei were of different forms, e.g. micronucleus, binucleate, multinucleate (Figure j–l). The most common form was cells with micronucleus and the other forms were with very low frequencies (Figure ).
3.2 Biochemical analysis
SDS-PAGE gel electrophoresis was carried out to monitor the change in gene expression of S. aureus and P. aeruginosa treated with AgNPs from their control (Figure ). The total number of bands per lane induced in S. aureus was ranging from 26 to 35 with molecular weight ranging from 90 to 14 kDa (Table a and b).
Figure 6. Change in protein profile of (a) Staphylococcus aureus and (b) Pseudomonas aeruginosa after treatment with silver nanoparticles.
(a) M: Marker; Sc: S. aureus control; S1%: S. aureus treated with 1% AgNPs; S3%: S. aureus treated with 3% AgNPs; S5%: S. aureus treated with 5% AgNPs.
(b) M: Marker; Pc: P. aeruginosa control; P1%: P. aeruginosa treated with 1% AgNPs;P3%: P. aeruginosa treated with 3% AgNPs; P5%: P. aeruginosa treated with 5% AgNPs.
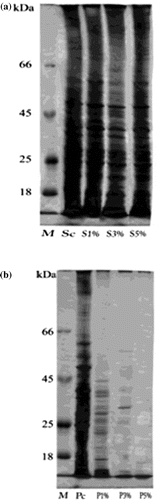
There were eight common protein bands present between the different strains of S. aureus. The molecular weights of them were 56, 51, 48, 38, 23, 21, 19 and16 kDa. Table a demonstrates the existence of 31 bands de novo induced after treatment with the three concentrations of AgNPs; some of them were specific to one dose of treatment. Only four bands completely disappeared after treatment with the three concentrations of AgNPs with molecular weights 82, 43, 36 and 14 kDa (Table a). The amount of expression of proteins in each treatment changed compared with the control, as can be observed by the changes in band intensity.
The protein profile of P. aeruginosa was obviously altered after treatment with AgNP suspension. The total number of bands decreased from 32 to two in a dose dependent manner. There were two common bands with molecular weights of 17 and 15 kDa, while 16 bands completely disappeared after AgNP suspension doses. Moreover, 14 bands were induced as a result of treatment with AgNP suspension; only three of them with molecular weights of 90, 33 and18 kDa were not dose specific. The band intensity of each treatment was changed as compared with their control (Table b).
3.3 Molecular analysis
RAPD-PCR and ISSR-PCR analyses are shown in Figures and respectively. The distributions of band products produced by different primers are shown in Tables and .
Figure 7. DNA polymorphism based on RAPD-PCR analysis of (a) Staphylococcus aureus and (b) Pseudomonas aeruginosa treated with silver nanoparticles.
(a) M: Marker; c: S. aureus control; T1: S. aureus treated with 1% AgNPs; T2: S. aureus treated with 3% AgNPs; T3: S. aureus treated with 5% AgNPs.
(b) M: Marker; c: P. aeruginosa control; T1: P. aeruginosa treated with 1% AgNPs; T2: P. aeruginosa treated with 3% AgNPs; T3: P. aeruginosa treated with 5% AgNPs.
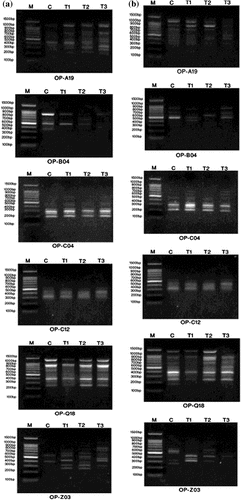
Figure 8. DNA polymorphism based on ISSR-PCR analysis of (a) Staphylococcus aureus and (b) Pseudomonas aeruginosa treated with silver nanoparticles.
(a) M: Marker; c: S. aureus control; T1: S. aureus treated with 1% AgNPs; T2: S. aureus treated with 3% AgNPs; T3: S. aureus treated with 5% AgNPs
(b) M: Marker; c: P. aeruginosa control; T1: P. aeruginosa treated with 1% AgNPs; T2: P. aeruginosa treated with 3% AgNPs; T3: P. aeruginosa treated with 5% AgNPs.
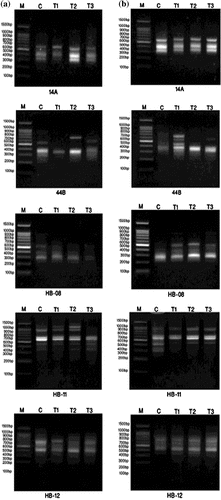
Table 1. Percentage of mitotic abnormalities and phase distribution of Allium cepa meristems treated with silver nanoparticles in different mitotic phases.
Table 2. Percentage of different types of abnormalities induced in Allium cepa meristems after treatment with silver nanoparticles.
Table 3a. SDS-PAGE electrophoretic profile of Staphylococcus aureus with intensities after treating with silver nanoparticles.
Table 3b. SDS-PAGE electrophoretic profile of Pseudomonas aeruginosa with intensities after treating with silver nanoparticles.
Table 4. RAPD-PCR and ISSR-PCR fragments, distribution of unique and polymorphic bands, monomorphic bands and percentage of polymorphism generated in Staphylococcus aureus treated with silver nanoparticles.
Table 5. RAPD-PCR and ISSR-PCR fragments, distribution of unique and polymorphic bands, monomorphic bands and percentage of polymorphism generated in Pseudomonas aeruginosa treated with silver nanoparticles.
The RAPD-PCR profile of S. aureus showed a total of 33 reproducible bands was amplified with the six different primers. There were eight polymorphic bands. The number of polymorphic bands varied between three and five. The average number of polymorphic fragments per primer was 1.3. The percentage of polymorphism ranged from 0% to maximum value of 87% with an average of 31.3% as shown in Table . One unique band was induced with primers OP-B04, OP-C04, while two unique bands were induced with OP-Z03, which produced the higher percentage of polymorphism, reaching 87.5%. The average percentage of polymorphism (31.3%) indicates the moderate level of polymorphism. In contrast, the RAPD-PCR profile of P. aeruginosa reveals a high level of polymorphism. The average percentage of polymorphism was 57%. There were 14 polymorphic bands of 39 generated fragments. Eight fragment bands were considered as unique bands. The OP-Q18 primer produces the maximum number of amplified fragments, attaining nine fragments; however the OP-C12 primer generates the minimum number of amplified fragments, achieving four fragments (Table ).
As shown in Table the total number of bands produced in S. aureus ISSR-PCR analysis was 27, ranging from four to six fragments with an average of 5.4 bands. Fifteen monomorphic bands with an average of three bands were found, while seven polymorphic bands with an average of 1.4 were induced. The percentage of polymorphism ranged from 16.7% to 66.7% with the average level of polymorphism reaching 43.7%. There were in total five unique bands with a mean of one unique band. There was no unique band produced by 14A and HB-11 primers, as can be seen in Table .
Regarding ISSR-PCR analysis of P. aeruginosa, the five primers yielded 29 highly reproducible ISSR fragments. HB-11 primer produced the maximum number of bands (nine bands), while the minimum number (four bands) was accomplished by both HB-08 and HB-12. Seven of the bands were polymorphic with an average of 1.4 polymorphic bands, while 13 bands were monomorphic. The percentage of polymorphism was found in the range of 0% (HB-12) to 75% (HB-08), with an average of 52.4%. Both HB-08 and HB-12 primers did not produce unique bands (Table ).
The cluster analysis was carried out to represent the genetic polymorphism in a graphic dendrogram. The UPGMA analysis based on RAPD-PCR and ISSR-PCR character difference was carried out with the three experimental groups and the control. There is a distinction into the two main clusters. One cluster is formed by the control strain and the other group is formed by the three treated groups. The treated cluster is split into two sub-clusters (Figure a and b). Only UPGMA analysis based on RAPD-PCR of S. aureus (Figure a1) represents the splitting of the experimental groups into two clusters. The first one consists of 5% AgNP-treated strains, while the second one represents the other two experimental groups and control.
4. Discussion
Recently there has been wide usage of AgNPs in several fields, therefore the present study has attempted to elucidate the hazards of AgNPs at the cellular level. Synthesis of (1, 3 and 5%) AgNPs were used to compare its effect against various types of cells. Two different eukaryotic models (human and plant) as well as one prokaryotic model (bacterial strains) were used to display a broad view of the toxic effect of AgNPs. The cytogenetic analysis carried out on A. cepa root tip cells and cytotoxic screening using human cells using MCF-7 and HepG2 cell lines revealed the cytotoxic and genotoxic effects of AgNPs. Meanwhile, the studies were extended to the biochemical and molecular levels of bacterial cells in order to broaden the investigation. Biochemical and molecular tests showed severe genetic variations displayed by protein electrophoresis, RAPD and ISSR-PCR tests.
The percentage viability of MCF-7 and HepG-2 tumour cell lines decreased with increased concentration of AgNPs. The concentration (dose) of AgNPs as well as cells used in the experiment plays a crucial role in the cytotoxic effect of AgNPs. AgNPs displayed cytotoxic chemotherapeutic activities, which could be used to target cancers with high proliferative rates (Gilman Citation1963; Kohn Citation1996). Many studies have demonstrated that cancer cells have a unique metabolism compared to normal cells (Herling et al. Citation2011). The cytotoxicity exerted by AgNPs in cancer cell lines is size-dependent, as smaller particles can enter cells easily (Liu et al. Citation2010). AgNP-induced cytotoxicity reduces cell viability in various cancer cell lines by causing apoptosis through the mitochondrial pathway and caspase 3 activation (Hsin et al. Citation2008; Gurunathan et al. Citation2013) and DNA fragmentation (Sriram et al. Citation2010), and generates reactive oxygen species, which are well known for causing lipid peroxidation of biological membranes and damage to structural proteins and DNA (Li and Osborne Citation2008). Toxicity of nanoparticles can be explained on the basis of a chemical toxicity based on the chemical composition, e.g. release of (toxic) ions and stress or stimuli caused by the surface, size and/or shape of the particles (Brunner et al. Citation2006). Therefore, the AgNPs herein may exert their toxicity through their physical character, which enhances production of ROS, causing DNA damage and interfering with cell cycle.
Additionally, our results showed that AgNPs exhibited cytotoxic potency by decreasing the mitotic index on the A. cepa in a dose-dependent manner. AgNPs could penetrate the plant system and may interfere with biological activity of the cell, causing cell damage (Kumari et al. Citation2009; Abdel-Azeem and Elsayed Citation2013) thus preventing a number of cells from entering mitosis and blocking the mitotic cycle (Rijstenbil et al. 1992), or extend the time of the G2 checkpoint to repair any DNA damage (Harrison and Haber Citation2006). Such cytostatic effects of AgNPs may be explained on the basis of the inhibition of nuclear protein synthesis required in the cell cycle (Kin and Bendixen Citation1997), or may be due to the inhibition of nucleic acids synthesis as observed in other plants treated with different heavy metals (El-Ghamery et al. Citation2002)
Reduction in the mitotic activity may also indicate the ability of AgNPs to inhibit or block the formation of various metabolites necessary for a normal sequence of mitosis. The present study proves the genotoxic effects of AgNPs in A. cepa, as well as cytotoxic effects in MCF-7 and HepG2 cell lines. Several studies including root exposure to high concentrations of different nanoparticles demonstrated NP phytotoxicity (Musante and White Citation2010; Yin et al. Citation2011; El-Temsah and Joner Citation2012; Ruffini Castiglioni et al. Citation2014).
The high prophase accumulation was previously noticed in treated A. cepa meristematic cells by Sobieh et al. (Citation2014). The prophase accumulation proves the toxic potency of a drug (Sobieh et al. Citation2014). Shehab (Citation1980) attributed the accumulation of A. cepa cells in prophase to a disturbance or breakdown of spindle apparatus. Moreover, Odeigah et al. (Citation1997) reported that, with increasing of treatment concentration and consequently increasing toxicity, there was an inhibitory effect on cell division. So, cells may be prevented from entering prophase or there may be prophase arrest where cells enter into mitosis but are arrested during prophase, resulting in a high frequency of prophase cells.
Various forms of chromosomal aberrations were detected in all phases of mitosis. The occurrence of stickiness reveals the irreversible genotoxic influence of AgNP suspension on A. cepa meristematic cells that leads to cell death (Liu et al. Citation1995). Stickiness is attributed to the difficulty of placement of inter chromosomal chromatin fibres or reflects the change in the properties of DNA or its structural protein (El-Ghamery et al. Citation2003).
Kabarity (Citation1966) suggested that the existence of irregular prophase could be due to the effect of treatment on the process of the individualization of chromatin threads to normal chromosomes, while the presence of the disturbance form may reflect the effect of AgNP suspension on microtubule polymerization and depolymerisation, leading to spindle disassembly. The formation of bridges can be attributed to chromosome stickiness, as elucidated by El-Ghamery et al. (Citation2003). Bridges can also result from the terminal deletion or loss of telomeres (Andraden et al. Citation2010). On the other hand, the occurrence of chromosome fragmentation indicates that not all the bridges are due to chromosome stickiness, but may be the result of breakage and reunion of the broken ends of chromosomes (El-Ghamery et al. Citation2003). The induction of fragments indicates the clastogenic potential of the AgNP suspension leading to a loss of genetic material (Raun and Lilum Citation1992). Several studies proved the cytotoxic and/or genotoxic effect of nanoparticles and the ability to induce various chromosomal aberrations in mammalian cells, bacteria and root meristematic cells of different plants (Patra et al. Citation2007; Kumari et al. Citation2009; Sahar et al. Citation2014).
The genotoxic effectiveness of the AgNP suspension was clearly detected by increasing micronucleus frequency. Micronuclei can originate from chromosome fragments, laggards or even a malfunctioning spindle (Grover and Kaur Citation1999). The AgNP suspension may form micronuclei by disturbing the spindle and inducing chromosome breaks. Micronuclei reveal a mutagenic effect, and they may lead to loss of the genetic material (Raun and Lilum Citation1992; Burroughs et al. Citation1996).
Electrophoretic analysis was performed to determine the effect of AgNPs on bacterial protein profile. Protein migration in this study demonstrated that nanoparticles caused changes in the protein profiles of P. aeruginosa more than S. aureus. Transmission electron microscope images reveal that the diameter of AgNPs inside P. aeruginosa was 9.11-10.2 nm, while those inside S. aureus were 14.5 nm (data not shown). It was proved that nanoparticles with a diameter less than 10 nm can attach to the sulphur-containing proteins on the bacterial cell wall, leading to increased permeability of the membrane, finally causing perforation of the bacterial cell wall as reported by Alexander et al. (Citation2008) and Li et al. (Citation2013). AgNPs also can release silver ions, which bind to biological macro-molecules, such as enzymes and proteins inside the bacterial cells (Guzman et al. Citation2012).
A combination of RAPD-PCR and ISSR-PCR analyses was used to investigate genetic polymorphism among control and AgNP-treated S. aureus strains and P. aeruginosa. RAPD-PCR and ISSR-PCR analyses detected genetic heterogeneity. Polymorphism was found in the presence and/or absence of DNA fragments between the samples. Atienzar and Jha (Citation2006) reported that the bands could disappear as a result of DNA damage, point mutations or chromosomal rearrangements induced by genotoxic compounds. AgNPs may cause damage to DNA by interacting with phosphorus groups of DNA molecules (Li et al. Citation2013). In addition, treatment with an AgNP suspension may decrease the replication ability of bacterial DNA and inactivate the cellular proteins (Feng et al. Citation2000). Silver ions released from AgNPs can interact with phosphorus groups in DNA, resulting in inactivation of DNA replication (Gupta and Silver Citation1998; Matsumura et al. Citation2003). So, changes in molecular response of P. aeruginosa and S. aureus after exposure to AgNPs may be correlated with structural rearrangements in bacterial DNA caused by different types of DNA damage or mutation.
UPGMA analysis was used to evaluate the genetic variation among different groups (Saitou and Nei Citation1987; Vannini et al. Citation2014). The present UPGMA data revealed genetic variability in treated strains as compared with control strain.
5. Conclusion
High concentrations of AgNPs had toxic effects in all the three biological systems analysed, i.e. cultured animal cells, plants (Allium cepa) and bacteria, and obstruct several intracellular components, causing damage and toxicity to cells. The induction of cytotoxicity in a human cell line by AgNPs, the antimitotic effect and the induction of genotoxic effects in A. cepa, as shown by a micronucleus assay, and the genetic variation observed in bacterial cells strengthen the attention when using high doses of AgNPs. However, further studies on the formation of protein coronas on the surface of AgNPs using FBS tests and evaluation of antioxidant enzymes and antioxidant compounds in the systems are needed.
Disclosure statement
No potential conflict of interest was reported by the authors.
Acknowledgements
The authors would like to thank Dr Mohamed Ahmed Betiha for supplying silver nanoparticle materials.
References
- Abdel-Azeem EA, Elsayed BA. 2013. Phytotoxicity of silver nanoparticles on Vicia faba seedlings. NY Sci J. 6(12):148–156.
- Ahmad A, Mukherjee P, Senapati S, Mandal D, Khan IM, Kumar R. 2003. Extracellular biosynthesis of silver nanoparticles using the fungus Fusarium oxysporum. Colloid Surf. B28(4):313–318.
- Alexander S, Klabunde KJ, George MR, Christopher MS. 2008. Biocidal activity of nanocrystalline silver powders and particles. Langmuir. 24(14):7457–7464.
- Andraden LF, Davide LC, Gedraite LS. 2010. The effect of cyanide compounds, fluorides, aluminum, and inorganic oxides present in spent potliner on germination and root tip cells of Lactuca sativa. Ecotox Environ Safe. 73(4):626–631.
- AshaRani PV, Mun GLK, Hande MP, Valiyaveettil S. 2009. Cytotoxicity and genotoxicity of silver nanoparticles in human cells. ACS Nano. 3(2):279–290.
- Atienzar FA, Jha AN. 2006. The random amplified polymorphic DNA (RAPD) assay and related techniques applied to genotoxicity and carcinogenesis studies: a critical review. Mutat Res. 613(2-3):76–102.
- Braydich-Stolle L, Hussain S, Schlager JJ, Hofmann MC. 2005. In vitro cytotoxicity of nanoparticles in mammalian germline stem cells. Toxicol Sci. 88(2):412–419.
- Brunner TJ, Wick P, Manser P, Spohn P, Grass RN, Limbach LK, Bruinink A, Stark WJ. 2006. In vitro cytotoxicity of oxide nanoparticles: comparison to asbestos, silica, and the effect of particle solubility. Environ Sci Technol. 40(14):4374.
- Burroughs BI, Johnson CS, Garry VF. 1996. In vitro micronucleus response of commercial chlorophenoxy herbicide and adjutants. Environ Mol Mutagen. 27:11.
- Carlson C, Hussain SM, Schrand AM, Braydich-Stolle LK, Hess KL, Jones RL, Schlager JJ. 2008. Unique cellular interaction of silver nanoparticles: sized pendent generation of reactive oxygen species. J Phys Chem B. 112(43):13608–13619.
- Choi O, Deng KK, Kim N Jr, Ross L, Rao YS, Hu Z. 2008. The inhibitory effects of silver nanoparticles, silver ions and silver chloride colloids on microbial growth. Water Res. 42(12):3066–3074.
- Dice LR. 1945. Measures of the amount of ecologic association between species. Ecology. 26(3):297–302.
- Elechiguerra JL, Burt JL, Morones JR, Camacho-Bragado A, Gao X, Lara HH, Yacaman MJ. 2005. Interaction of silver nanoparticles with HIV-1. J Nanobiotechnol. 3:6. doi: 10.1186/1477-3155-3-6.
- El-Ghamery AA, El-Kholy MA, Abou El-Yousser MA. 2003. Evaluation of cytological effects of Zn2+ in relation to germination and root growth of Nigella sativa L. and Triticum aestivum L. Mutat Res. 537(1):29–41.
- El-Ghamery AA, Mansour MM, Abou El-Yousser MA. 2002. Effect of some heavy metals on mitotic activity, nucleic acids content and protein banding patterns in meristematic roots of Nigella sativa and Triticum aestivum. Egypt J Biotechnol. 11:266–281.
- El-Temsah YS, Joner EJ. 2012. Impact of Fe and Ag nanoparticles on seed germina-tion and differences in bioavailability during exposure in aqueous suspension and soil. Environ Toxicol. 27(1):42–49.
- Feng QL, Wu J, Chen GQ, Cui FZ, Kim TN, Kim JO. 2000. A mechanistic study of the antibacterial effect of silver ions on Escherichia coli and Staphylococcus aureus. J Biomed Mater Res. 52(4):662–668.
- Fiskesjo G. 1997. Allium test for screening chemicals, evaluation of cytological parameters. In: Wang W, Gorsuch JW, Hughes JS, editors. Plants for environmental studies. Boca Raton, New York: CRC Lewis p; p. 308.
- Gilman A. 1963. The initial clinical trial of nitrogen mustard. Am J Surg. 105(5):574–578.
- Grover IS, Kaur S. 1999. Genotoxicity of waste water samples from sewage and industrial effluent detected by the Allium root anaphase aberration and micronucleus assays. Mutat Res. 426(2):183–188.
- Gupta A, Silver S. 1998. Silver as a biocide: will resistance become a problem? Nature Biotechnol. 16:888–890.
- Gurunathan S, Raman J, Abd Malek SN, John PA, Vikineswary S. 2013. Green synthesis of silver nanoparticles using Ganoderma neo-japonicum Imazeki: a potential cytotoxic agent against breast cancer cells. Int J Nanomed. 8(1):4399–4413.
- Guzman M, Dille J, Godet S. 2012. Synthesis antibacterial activity of silver nanoparti-cles against gram-positive and gram-negative bacteria. Nanomed Nanotechnol Biol Med. 8(1):37–45.
- Harley JP, Prescott LM. 2002. Laboratory exercises in microbiology. 5th ed. New York, NY: The McGraw-Hill.
- Harrison JC, Haber JE. 2006. Surviving the breakup: the DNA damage checkpoint. Annu Rev Genet. 40:209–235.
- Herling A, Konig M, Bulik S, Holzhutter HG. 2011. Enzymatic features of the glucose metabolism in tumor cells. Fed Eur Biochem Soc J. 278(14):2436–2459.
- Hsin Y, Chen C, Huang S, Shih T, Lai P, Chueh P. 2008. The apoptotic effect of nanosilver is mediated by ROS- and JNK-dependent mechanism involving the mitochondrial pathway in NIH3T3 cells. Toxicol Lett. 179(3):130–139.
- Hussain SM, Hess KL, Gearhart JM, Geiss KT, Schlager J. 2005. In vitro toxicity of nanoparticles in BRL 3A rat liver cells. Toxicol In Vitro. 19(7):975–983.
- Kabarity A. 1966. The effect of certain mutagenic substances upon prophase. Beitr-Bit. Pflanzen. 42:317–326.
- Kaveh R, Li YS, Ranjbar S, Tehrani R, Brueck CL, Van Aken B. 2013. Changes in Arabidopsis thaliana gene expression in response to silver nanoparticles and silver ions. Environ Sci Technol. 47(18):10637–10644.
- Kheiralla ZMH, Rushdy AA, Betiha MA, Yakob NA. 2014. High-performance antibacterial of montmorillonite decorated with silver nanoparticles using microwave-assisted method. J Nanopart Res. 16:2560–2566.
- Kim S, Choi JE, Choi J, Chang KH, Park K, Yi J, Ryu DY. 2009. Oxidative stress-dependent toxicity of silver nanoparticles in human hepatoma cells. Toxicol In Vitro. 23(6):1076–1084.
- Kim YK, Lee YS, Jeong DH, Cho MH. 2007. Antimicrobial effect of silver nanoparticles. Nanomedicine. 3(1):95–101.
- Kin JC, Bendixen EL. 1997. The effect Halooxyfob and CGA-82725 on cell cycle and cell division of oat (Avena sativa) root tips. Weed Sci. 35(6):769–774.
- Kohn KW. 1996. Beyond DNA cross-linking: history and prospects of DNA-targeted cancer treatment –fifteenth Bruce F. Cain Memorial Award Lecture. Cancer Res. 56:5533–5546.
- Kumar C. 2006. Nanomaterials. Toxicity, health and environmental issues. Weinheim: Wiley.
- Kumari M, Khan SS, Pakrashi S, Mukherjee A, Chandrasekaran N. 2011. Cytogenetic and genotoxic effects of zinc oxide nanoparticles on root cells of Allium cepa. J Hazard Mater. 90(1):613–621.
- Kumari M, Mukherjee A, Chandrasekaran N. 2009. Genotoxicity of silver nanoparticles in Allium cepa. Sci Total Environ. 407(19):5243–5246.
- Laemmli UK. 1970. Cleavage of structural proteins during the assembly of the head of bacteriophage T4. Nature. 227(5259):680–685.
- Leme DM, Marine-Morales MA. 2009. Allium cepa test in environmental monitoring: a review on its applications. Mutat Res. 682(1):71–81.
- Li GY, Osborne NN. 2008. Oxidative-induced apoptosis to an immortalized ganglion cell line is caspase independent but involves the activation of poly (ADP-ribose) polymerase and apoptosis-inducing factor. Brain Res. 188:35–43.
- Li LH, Yen MY, Ho CC, Wu P, Wang CC, Maurya PK, Chen PS, Chen W, Hsieh WY, Chen HW. 2013. Non-cytotoxic nanomaterials enhance antimicrobial activities of cefmetazole against multidrug-resistant Neisseria gonorrhoeae. PLoS One. 8(5):1–10.
- Liu DH, Jiang WS, Wang W, Zhai L. 1995. Evaluation of metal ion toxicity on root tip cells by the Allium test. Israel J Plant Sci. 43:125–133.
- Liu W, Wu Y, Wang C, Li HC, Wang T, Liao CY, Cui L, Zhou QF, Yan B, Jiang GB. 2010. Impact of silver nanoparticles on human cells: effect of particle size. Nanotoxicology. 4(3):319–330.
- Ma TH, Xu Z, Xu C, McConnell H, Rabago EV, Arreola GA, Zhang H. 1995. The improved Allium/Vicia root tip micronucleus assay for clastogenicity of environmental pollutants. Mutat Res. 334(2):185–195.
- Matsumura Y, Yoshikata K, Kunisaki S, Tsuchido T. 2003. Mode of bactericidal action of silver zeolite and its comparison with that of silver nitrate. Appl Environ Microbiol. 69(7):4278–4281.
- Mosmann T. 1983. Rapid colorimetric assay for cellular growth and survival: application to proliferation and cytotoxicity assays. J Immunol Method. 65(1–2):55–63.
- Musante C, White JC. 2010. Toxicity of silver and copper to Cucurbita pepo: differential effects of nano and bulk-size particles. Environ Toxicol. 27(9):510–517.
- Navarro E, Piccapietra F, Wagner B, Marconi F, Kaegi R, Odzak N, Sigg L, Behra A. 2008. Toxicity of silver nanoparticles to Clamydomonas reinhardtii. Environ Sci Technol. 42(23):8959–8964.
- Oberdörster G. 1996. Effects of ultrafine particles in the lung and potential relevance to environmental particles. In: Marijnissen JMC, Gradon L, editors. Aerosol Inhalation. Dordrecht: Kluwer Academic; p. 165.
- Odeigah PGC, Nurudeen O, Amund OO. 1997. Genotoxicity of oil field wastewater in Nigeria. Hereditas. 126(2):161–167.
- Patlolla AK, Berry A, May L, Tchounwou PB. 2012. Genotoxicity of silver nanoparticles in Vicia faba: a pilot study on the environmental monitoring of nanoparticles. Int J Environ Res Publ Health. 9(5):1649–1662.
- Patra HK, Banerjee S, Chaudhuri U, Lahiri P, Dasgupta AK. 2007. Cell-selective response to gold nanoparticles. Nanomedicine. 3(2):111–119.
- Pulate PV, Ghurde MU, Deshmukh VR. 2011. Cytological effects of the biological and chemical silver-nanoparticles in Allium cepa L. Int J Innov Bio-Sci. 1:32–35.
- Ramachandran V, Ismail P, Stanslas J, Shamsudin N. 2009. Analysis of renin-angiotensin aldosterone system gene polymorphisms in Malaysian essential hypertensive and type 2 diabetic subjects. Cardiovasc Diabet. 8:11. doi:10.1186/1475-2840-8-11.
- Raun C, Lilum J. 1992. Application of micronucleus test in Vicia faba root tips in the rapid detection of mutagenic environmental pollutants. Chin J Environ Sci. 4(1):56–58.
- Rijstenbil JW, Poortvliet TCW. 1992. Copper and zinc in estuarine water: chemical speciation in relation to bioavailability to the marine planktonic diatom Ditylum brightwellii. Environ Toxicol Chem. 11(11):1615–1625.
- Ruffini Castiglione M, Giorgetti L, Cremonini R, Bottega S, Spanò C. 2014. Impact of TiO2 nanoparticles on Vicia narbonensis L.: potential toxicity effects. Protoplasma. 251(6):1471–1479.
- Sahar AT, Zakia MA, Sobieh ShS. 2014. Assessment of Punica granatum L. extract on the mitotic arrest of plant bioassay system. Life Sci J. 11(8):757–770.
- Saitou N, Nei M. 1987. The neighbor-joining method: a new method for reconstruction phylogenetic trees. Mol Biol Evol. 4(4):406–442.
- Sammons DW, Adrune LD, Nishizawa EE. 1981. Ultrasensitive silver-based color staining of polypeptides in polyacrylamide gels. Electrophoresis. 2(3):135–141.
- Samuel U, Guggenbichler JP. 2004. Prevention of catheter-related infections: the potential of a new nano-silver impregnated catheter. Int J Antimicrob Agent. 23(1):S75–78.
- Shahverdi AR, Fakhimi A, Shahverdi HR, Minaian S. 2007. Synthesis and effect of silver nanoparticles on the antibacterial activity of different antibiotics against Staphylococcus aureus and Escherichia coli. Nanomedicine. 3(2):168–71.
- Shehab AS. 1980. Cytological effects of medicinal plants in Qatar 11.Mitotic effect of water extract of Teucrium pilosum on Allium cepa. Cytologia. 45(1-2):57–64.
- Sobieh ShS, Tawab SA, Fahmy DM. 2014. Deregulation of mitosis progression and cytotoxic effect triggered in Allium cepa L. roots by Rubus sancatus Schreber extract. Life Sci J. 11(11):1047–1058.
- Sondi I, Salopek-Sondi B. 2004. Silver nanoparticles as antimicrobial agent: a case study on E. coli as a model for Gram-negative bacteria. J Colloid Interface Sci. 275(1):177–182.
- Sriram MI, Kanth SB, Kalishwaralal K, Gurunathan S. 2010. Antitumor activity of silver nanoparticles in Dalton’s lymphoma ascites tumor model. Int J Nanomed. 5(1):753–762.
- Theng BK. 1974. The chemistry of clay-organic reactions. London: Adam Hilger.
- Vannini C, Domingo G, Onelli E, Prinsi B, Marsoni M, Espen L, Bracale M. 2013. Morphological and proteomic responses of Eruca sativa exposed to silver nanoparticles or silvernitrate. PLoS ONE. 8(7):1–8.
- Vannini C, Domingoa G, Onellib E, De Mattiac F, Brunic I, Marsonia M, Bracale M. 2014. Phytotoxic and genotoxic effects of silver nanoparticles exposure on germinating wheat seedlings. J Plant Physiol. 171(13):1142–1148.
- Williams JGK, Kubelk AR, Livak KJ, Rafalski JA, Tingey VA. 1990. DNA polymorphisms amplified by arbitrary primers are useful as genetic markers. Nucl Acid Res. 18(22):6231–6235.
- Yin LY, Cheng YW, Espinasse B, Colman BP, Auffan M, Wiesner M, Rose J, Liu J, Bernhardt ES. 2011. More than the ions: the effects of silver nanoparticles on Lolium multiflorum. Environ Sci Technol. 45(6):2360–2367.
- Zietkiewicz E, Rafalski A, Labuda D. 1994. Genome finger-printing by simple sequence repeats (SSR) anchored polymerase chain reaction amplification. Genomics. 20(2):176–183.